Full Text View
Volume 23 Issue 2 (February 2013)
GSA Today
Article, pp. 4-10 | Abstract | PDF (5.2MB)
Miocene rejuvenation of topographic relief in the southern Appalachians
Table of Contents |
---|
Search GoogleScholar for
Search GSA Today ![]() |
![]() |
Abstract
Conventional wisdom holds that the southern Appalachian Mountains have not experienced a significant phase of tectonic forcing for >200 myr; yet, they share many characteristics with tectonically active settings, including locally high topographic relief, steep slopes, incised river gorges, and frequent mass-wasting events. Two competing hypotheses are commonly used to explain their modern topographic expression. One suggests that relief is largely controlled by variable lithologic resistance to weathering and that their modern form has long persisted in a dynamic equilibrium. The second postulates that their relief is a product of recent rejuvenation, driven either by climate change or the epeirogenic uplift of the land surface driven by mantle forcing. Within portions of the Cullasaja River basin of the southern Appalachians, we show that relief has increased by >150% since the Miocene. Evident within the basin are a set of retreating knickpoints that delineate a rugged, actively incising landscape from lower-relief relict topography. Constraints on the timing of knickpoint entry into the basin suggest that the process of landscape rejuvenation began well prior to the late Cenozoic (<4 myr) transition to a more oscillatory (glacial-interglacial) climate regime. Furthermore, the geomorphology of the Cullasaja River basin is difficult to reconcile in the context of a transition to a more erosive climatic regime but is consistent with an epeirogenically uplifted landscape. Consequently, these observations lend new support to the idea that the rugged topography of the southern Appalachians has developed in response to post-orogenic regional uplift in the Miocene.
*Email:
Manuscript received 10 Aug. 2012; accepted 30 Oct. 2012
DOI: 10.1130/GSATG163A.1.
Introduction
Topographic relief exerts an essential control on the rates and processes involved in landscape denudation (Ahnert, 1970; Montgomery and Brandon, 2002), influencing feedbacks between atmospheric, earth-surface and rock exhumation processes, variations in sediment flux, and the magnitude and style of gravity-driven natural hazards. A long-standing debate in the geosciences is centered upon the nature of topographic decay in post-orogenic mountain ranges (Davis, 1889; Hack, 1960; Bishop, 2007). Central to this debate are the still-rugged terrains within the modern Appalachians Mountains of eastern North America, where the last significant phase of tectonic activity presumably ceased shortly after Late Triassic rifting of the Atlantic margin (Hatcher, 1989).
Two hypotheses have been put forth to explain the occurrence of locally high topographic relief, steep slopes, incised river gorges, and frequent mass-wasting events along the passive margin of the southern Appalachians (e.g., Gallen et al., 2011; Wooten et al., 2008). One suggests that topography has persisted though time in a dynamic equilibrium, with relief largely controlled by the variable erodibility of rock units (Hack, 1960; Matmon et al., 2003). The second posits that modern relief is a product of recent rejuvenation (Hack, 1982); however, whether the process governing this resurgence is climate change (Molnar, 2004; Hancock and Kirwan, 2007) or dynamic mantle processes forcing epeirogenic uplift (Pazzaglia and Brandon, 1996) is debated. Recent results obtained from the application of thermochronology (Boettcher and Milliken, 1994) and terrestrial cosmogenic radionuclides (CRNs; Matmon et al., 2003; Hancock and Kirwan, 2007) have not led to a consensus regarding the processes governing the evolution of relief within this landscape—a result of contrasting interpretations drawn from different datasets.
We test the competing hypotheses of dynamic equilibrium and topographic rejuvenation with a study of the geomorphology of the ~300 km2 Cullasaja River basin of the southern Appalachian Mountains in western North Carolina (Figs. 1A and 1B). The Cullasaja is a tributary to the Little Tennessee River, its waters traveling >1500 river kilometers before discharging into the Gulf of Mexico (Fig. 1A). The timing and magnitude of changes in relief within the basin are quantified through the analysis of a 6-m horizontal resolution LiDAR elevation dataset. Results indicate that the Cullasaja basin landscape has undergone a period of rejuvenation, with relief increasing >150% since the Miocene. The timing of this rejuvenation and the geomorphic expression of the Cullasaja basin landscape, however, suggest that climate change is not the fundamental driving process (cf. Molnar, 2004). Rather, observational evidence favors a model where relief develops as the landscape is epeirogenically uplifted.
(A) Shaded relief map of the southern Appalachians of western North Carolina and eastern Tennessee, USA. BRE (black dashed line)—Blue Ridge Escarpment; GSM (gray dashed oval)—Great Smoky Mountains. Inset map shows the location of this region in the context of the eastern continental divide (ECD) and the southern limit of glaciation during the last glacial maximum (LGL) (Thelin and Pike, 1991). The headwaters of the Cullasaja River basin (CRB) are >1500 km from the outlet of the Mississippi River. (B) Shaded relief image of the Cullasaja basin with the position of the 44 knickpoints identified in this study. (C) Histogram of knickpoint elevations in 25 m bins. Yellow stars denote the elevations of the trunk channel knickpoints identified in D. (D) Longitudinal river profiles of 52 streams showing the location of 44 knickpoints, relict reaches, and the approximate transition between fluvial and colluvial/debris flow–dominated channels that occurs at drainage areas ≥1.25 × 105 m2. Red dots—knickpoints; green dots—highest knickpoints |
Study Area
The Cullasaja River basin contains the geomorphic features required to reconstruct its paleo-relief, including numerous active river knickpoints—sharp convexities in an otherwise concave-up longitudinal river profile—and a preserved relict landscape “surface.” The study area lacks evidence of late Pleistocene glaciation and because of its distance from the maximum extent of late Quaternary ice sheets (Thelin and Pike, 1991), the Cullasaja basin experienced little, if any, glacial isostatic response (Fig. 1A). This is supported by studies using decade-long continuous GPS and satellite gravity surveys to estimate vertical motions due to glacial isostatic adjustments and marine flooding of the North American continental shelf. These datasets demonstrate that the southern Appalachians are either a null region or are slowly subsiding at rates <0.3 mm yr−1 (Sella et al., 2007; Wu et al., 2010).
The Cullasaja basin is a detachment-limited geomorphic system with bedrock channels flowing across mostly high-grade gneisses (Gallen et al., 2011) (Fig. DR1, GSA supplemental data repository 1 ). A distinct break in log-log channel slope versus upstream drainage area scaling is interpreted to represent the transition between debris flow and fluvial-dominated channels (cf. Montgomery and Foufoula-Georgiou, 1993; Wobus et al., 2006). The maximum drainage area where this scaling break was observed in the Cullasaja basin is ~1.25 × 105 m2. We choose this as the minimum contributing area in defining the fluvial network. Three observations suggest that lithologic control on hillslope erosion and river incision is relatively uniform throughout the basin: (1) most river channels do not follow lithologic contacts; (2) channel steepness shows no obvious correlation to rock-type; and (3) the observed location of fluvial knickpoints is generally discordant with lithologic contacts (Gallen et al., 2011) (Fig. DR1B [see footnote 1]).
1 GSA supplemental data item 2013103, supplemental information, definitions, figures, tables, and references, is online at www.geosociety.org/pubs/ft2013.htm. You can also request a copy from GSA Today, P.O. Box 9140, Boulder, CO 80301-9140, USA; .
Tributary knickpoints cluster within five altitudinal bands that are coincident with the elevations of five prominent main-stem knickpoints (Figs. 1C and 1D). We appeal to a kinematic model of active knickpoint retreat (e.g., Niemann et al., 2001), which predicts uniformity in vertical velocity for knickpoints resulting from a common base level fall. We interpret the knickpoint clusters as independent waves of bedrock incision actively propagating though the drainage network. The process(es) responsible for knickpoint initiation is unknown; however, eustatic fluctuations are an unlikely mechanism, as it has been shown that such signals do not propagate beyond the lower alluvial reaches of the Mississippi River (Schumm, 1993). Furthermore, the size of knickpoints identified (gradients ≥0.1, dropping >20 m), the total amount of knickpoint relief in the Cullasaja basin (>400 m), and the absence of localities for large-magnitude stream capture events or deep-seated rockslides preclude autogenic knickpoint formation (cf. Wooten et al., 2008; Korup et al., 2006; Prince et al., 2011). With no obvious mechanism for generating the knickpoints, we assess the paleo-topographic conditions in the basin to test the hypothesis that the knickpoints represent a change in geomorphic boundary conditions external to the Cullasaja basin and attempt to determine when this transition began. In doing so we aim to clarify the process(es) driving landscape evolution in the southern Appalachians through the generation of these knickpoints.
Dynamic Equilibrium Versus Topographic Rejuvenation
The highest flight of 11 knickpoints demarcates an important topographic transition in the Cullasaja basin; downstream of the knickpoints, local relief, hillslope and stream channel steepness, and the frequency of landslides all increase significantly when compared to the portion of the landscape isolated above the knickpoints (>1150 m) (Gallen et al., 2011) (Figs. 2A–2D; Table DR1 [see footnote 1]). The occurrence of the knickpoints across a spread in drainage areas (1.4 × 105–7.5 × 106 m2) implies that they are not stalled at a threshold drainage area, an assumption later tested with numerical modeling (i.e., Crosby and Whipple, 2006; Berlin and Anderson, 2007). Rather, the highest set of knickpoints defines the propagating front of river incision, representing the boundary between an upper-relict landscape and a lower-actively adjusting zone (Fig. 2D) (Clark et al., 2005). This evidence has two important implications: (1) the Cullasaja basin, and probably the entirety of the southern Appalachians, is in a transient state of adjustment, rather than a dynamic equilibrium (cf. Hack, 1960; Matmon et al., 2003), where topography is rejuvenated in the passing wake of mobile knickpoints; and (2) the highest knickpoints and the relict landscape that they isolate contain information about the onset of enhanced incision and the temporal evolution of topography in this region.
Topographic and fluvial metrics and characterization of relict surface. (A and B) Maps of local (A) slope and (B) relief for the Cullasaja River basin. (C) Normalized channel steepness (ksn) averaged every 100 m along each stream reach. (D) Perspective view of the Cullasaja River basin highlighting the relict reach (shaded) preserved above the highest flight of knickpoints. (E) Plot showing Ahnert’s (1970) global relationship between mean relief and mean denudation and the estimated mean denudation rates of the active and relict portions of the Cullasaja River basin with 1s errors as determined in mean local relief calculations. |
Estimating Rates of Erosion
Ahnert (1970) observed that mean local relief in temperate mid-latitude drainage basins from tectonically inactive settings scales linearly with mean denudation rate. Application of Ahnert’s relationship to the Cullasaja basin suggests that erosion rates, based on calculations of mean local relief, are 27 ± 11 and 6 ± 6 mm kyr−1 in the active and relict portions of the landscape, respectively (Fig. 2E; Table DR1). A slower rate of erosion for the relict landscape is supported by a reduction in landslide occurrence (Wooten et al., 2008) (Fig. DR1; Table DR1 [see footnote 1]) and an increase in mean soil thickness (Thomas, 1996) relative to the active landscape. These estimates closely align with regional 10Be CRN studies; the active landscape erosion rate matches basin average rates from the Great Smokey Mountains of 27 ± 4 mm kyr−1 (Matmon et al., 2003), and the relict landscape prediction is consistent with West Virginia bedrock summit lowering rates (6 ± 3 mm kyr−1) (Hancock and Kirwan, 2007), indicating that they are reasonable values.
Paleo-Relief Reconstructions
To estimate the magnitude of paleo-relief in the Cullasaja basin, we first determined the paleo–base level of the relict surface using the channel segments above the highest flight of knickpoints. Equilibrium longitudinal river profiles of the relict channel reaches are reconstructed using the empirically derived scaling law that relates local channel slope (S) to drainage area (A) through the channel parameters of steepness (ks) and concavity (θ) (e.g., Flint, 1974):
S = ksA−θ. (1)
Of the 11 reaches analyzed, eight had sufficient data to determine estimates of channel steepness and concavity (Fig. DR2; Tables DR2 and DR3 [see footnote 1]). To avoid geomorphic and hydrologic complications introduced at a smaller drainage area, channel steepness indices (ksn) were normalized using the mean concavity (θref) of the eight reaches (Table DR3) (Clark et al., 2005; Wobus et al., 2006).
The elevations of the reconstructed tributary and trunk channel profiles fall within error at their confluences and are therefore graded to the same paleo–base level that is ~480 m higher than the present-day river mouth (Figs. 3 and DR2). Assuming that the ridge line erosion rates determined in West Virginia (Hancock and Kirwan, 2007) are regionally applicable to the southern Appalachians implies that the vertical distance between the ridge lines and the relict landscape of the Cullasaja basin has remained approximately the same through time. Paleo-relief in the relict landscape is thus determined to be ~300 ± 25 m by differencing the elevations of the reconstructed river profile from the modern-day drainage divide (Fig. 3). This estimation suggests that relief in the Cullasaja basin has increased 163% ± 24% since the highest knickpoints entered the mouth of the Cullasaja River (Fig. 3).
Modern and reconstructed paleo-river profiles with the modeled elevations of tributary-trunk channel junctions projected to the paleo profile. The 2σ elevation errors are from the normalized steepness indices and are based on linear regressions through log-log channel slope-drainage area data (Fig. DR2 [see text footnote 1]). The amount of paleo-relief in the Cullasaja River basin is based on the assumption that the ridge lines have eroded at a rate commensurate with the mean denudation rate of the relict surface (6 ± 6 mm kyr−1). |
Timing of Topographic Resurgence
The time that the highest trunk channel knickpoint, Highland Falls, passed the mouth of the Cullasaja basin represents a minimum age for the relict landscape and hence the initiation of newly imposed geomorphic boundary conditions. To our knowledge, there are no preserved fluvial terraces related to the upper-most knickpoints in the Cullasaja basin, eliminating more conventional methods for determining their age and propagation rates. Instead, a simple yet novel approach is used to constrain the timing of knickpoint entry into the basin. Assuming that knickpoint propagation proceeded as a kinematic wave, the travel time of the highest knickpoints from the river mouth to their current position is the same as the time required to erode the rock volume “missing” from the active landscape (Fig. 4A) (Norton et al., 2008). The knickpoint travel time (tk) is estimated by:
, (2)
where Acand V are the area below the knickpoint elevation contour and volume, respectively, and is the average erosion rate within the active landscape (Fig. 4A). A minimum estimate of the volume of rock eroded from beneath the highest knickpoints is found by differencing the basin topography with a sloping surface fit to the modern drainage divide, and a maximum estimate is determined by differencing the basin topography with a horizontal surface defined by the elevation contour at the top of Highland Falls (Fig. 4B).
Conceptual model and results of volume-for-time substitutions. (A) Cartoon illustrating the idealized evolution of a drainage basin experiencing base-level lowering brought on by the propagation of a knickpoint as a kinematic wave. The final time step (t2) identifies the volume of eroded material (V) and area of the active landscape (Ac), which are the parameters used to calculate the time since a knickpoint entered the mouth of a drainage basin using equation 2. (B) Schematic cross section illustrating the two methods used to estimate “missing” volume below the elevation of the highest trunk channel knickpoint. (C) Plot of the estimated time since the Highland Falls knickpoint entered the mouth of the Cullasaja River basin. The vertical black line is the mean basin average erosion rate determined for the active portion of the Cullasaja River basin predicted by the Ahnert (1970) trend (27 ± 11 mm kyr−1) and matches that of the nearby Great Smokey Mountains (Matmon et al., 2003; 27 ± 4 mm kyr−1). |
Using estimates of the basin average erosion rate for the active landscape between 16 and 38 mm kyr−1 (Figs. 2E and 4C), Highland Falls would have entered the mouth of the river between 17.6 and 4.6 Ma. Applying the mean denudation rate and average volume of eroded rock, the entry time becomes ca. 8.5 Ma (Fig. 4C). It is, however, unlikely that these knickpoints were formed at the mouth of the Cullasaja basin. Even if the Cullasaja River was not incising (0 mm kyr−1), while the Little Tennessee River cut down at 600 mm kyr−1 (estimated from the fastest rates of river incision measured in Appalachian draining rivers by Reusser et al., 2004), such conditions would need to be sustained >150 kyr to form the largest Cullasaja basin knickpoints (>100 m). The long-term persistence of such conditions is unrealistic; rather, the knickpoints likely formed some distance down the Little Tennessee River by some other mechanism. This 17.6–4.6 Ma time range therefore provides an extreme minimum value for the age of the relict surface, and thus the onset of modern relief production in the southern Appalachians almost certainly pre-dates the transition to significant orbitally driven climate unsteadiness beginning 4 to 3 Ma (Peizhen et al., 2001; Molnar, 2004).
Moreover, it is difficult to explain the geomorphology of the Cullasaja basin in the framework of climate change. A transition to a cooler, wetter, and rapidly fluctuating climate should enhance regional erosional efficiency (Molnar, 2004), but it would need to be locally absent in order to preserve a relict surface, which is highly unlikely in the Appalachians. A change to a more erosive environment also is predicted to reduce channel steepness (Whipple and Tucker, 1999; Wobus et al., 2010), not increase it as is observed in the Cullasaja basin. Collectively, the timing of topographic rejuvenation and geomorphology of the Cullasaja basin eliminates late Cenozoic (<4 myr) climate change as the fundamental driver of the enhancement of relief in the southern Appalachians.
Testing Key Assumptions
We numerically model the spatial distribution of the 11 highest knickpoints in the Cullasaja basin to test the assumptions that the knickpoints are: (1) genetically related and (2) verify that they are still actively propagating and not stalled. Testing these assumptions is important because it will show that the uppermost knickpoints originated from a single source and are currently moving through and dissecting the relict landscape. Further, modeling will support the age constraints from above if knickpoint velocity is determined to be reasonable (e.g., within measured values). To this end, a generic knickpoint celerity model is used (Crosby and Whipple, 2006):
, (3)
where dx/dt is the upstream knickpoint migration rate in m yr−1, C is a dimensional coefficient of erodability in units m(1–2p) yr−1, A is contributing drainage area, and p is a non-dimensional constant reflecting knickpoint celerity dependence on drainage area, a proxy for discharge. A brute-force two-parameter search is used to find the best-fitting C and p parameters that minimize the misfit between the observed and modeled knickpoint positions (Crosby and Whipple, 2006; Berlin and Anderson, 2007).
Using the results from the volume-for-time substitutions (eq. 2), we consider a suite of 28 model runs with knickpoints entering the Cullasaja basin between 4.5 to 18 Ma (0.5 myr intervals). Each model run worked equally well, with <2% difference in the sum of the least squares residual between the observed and modeled knickpoint positions for any given travel time (Figs. DR3 and DR4 [see footnote 1]). These models predict the position of the 11 highest knickpoints remarkably well (Figs. 5A and 5B), implying that the knickpoints do behave as a kinematic wave. Present-day minimum knickpoint velocity varies between 0.13 and 2.25 mm yr−1 for the set of modeled travel times, confirming that the knickpoints are mobile and dissecting the relict landscape. Best fitting p parameters ranging from 0.51 to 0.54 are consistent with a square root of area scaling (Berlin and Anderson, 2007) (Fig. DR3). The erosional coefficient C, however, adjusts over nearly an order of magnitude to accommodate the modeled knickpoint travel time (Figs. DR3 and DR4). Nonetheless, the maximum and mean knickpoint velocity predicted by the numerical modeling are within the range of measured knickpoint propagation rates (Loget and Van Den Driessche, 2009) (Fig. 5C), suggesting that calculated knickpoint travel times are reasonable. Independent support for reasonable knickpoint travel times comes from estimates of river incision that are within the range of measured long-term (104–106 yr) valley incision for Appalachian-draining rivers (Mills, 2000; Reusser et al., 2006) that, based on our longitudinal profile reconstructions (Fig. 3), fall between 24 and 140 mm kyr−1.
Knickpoint celerity model results. Example of results from the best-fitting C and p parameters for a knickpoint travel time of 8.5 myr, which is the mean time prediction based upon the estimated basin average erosion rate from the Ahnert (1970) relationship as well as the average of the two volume-for-time estimates (see Fig. 4). (A) Observed and modeled knickpoint distribution. Mean error refers to the mean of the differences between the observed knickpoint and the modeled knickpoint distance from the mouth of the Cullasaja River. (B) Relationship between observed and modeled knickpoint (kp) positions, expressed with respect to their distance from the mouth (dfm) of the Cullasaja River. (C) Maximum and mean (gray is ±1σ error) velocity of the best fitting model result associated with each run. |
Driving Mechanism For Topographic Rejuvenation
The proposed timing of the topographic resurgence reported here is roughly concurrent with previous studies that demonstrate increased sedimentation rates and grain sizes delivered from the Appalachians eastward to off-shore basins along the Atlantic margin (Poag and Sevon, 1989; Pazzaglia and Brandon, 1996) and westward to the Mississippi embayment (Potter, 1955) and Gulf of Mexico (Galloway et al., 2011) ca. 16 to 12 Ma. This coincidence suggests a causative link between the process responsible for relief rejuvenation in the Appalachians and the flux of sediment to adjacent depocenters and implies a regional disturbance to the Appalachians in the Miocene. With no obvious surficial process driving the Miocene topographic resurgence, what mechanism can be called upon to explain the results reported in this paper? The formation of large knickpoints, the steepening of river gradients, the ongoing dissection of a relict landscape, and the pulse of sediment to offshore basins are broadly consistent with a region that has undergone uplift; however, the Appalachians generally lack evidence of late Cenozoic deformation (Hatcher, 1989).
Epeirogenic uplift of the southern Rocky Mountains and Colorado Plateau (Karlstrom et al., 2012) and the southern Sierra Nevada range (Clark et al., 2005) has produced a similar geomorphic response to what is reported here. In these settings, the uplifted regions also exhibit strong spatial correlations with geophysical anomalies in the crust and lithospheric mantle, providing insight into the driving mechanism(s). Although large-scale geophysical imaging of the tectonically passive eastern United States has received relatively little attention compared to the western United States, Grand et al. (1997) and Ren et al. (2007) have documented fragments of the relict Farallon oceanic slab within the mantle beneath the modern Appalachians. More recently, Wagner et al. (2012) produced receiver function profiles crossing the southern Appalachians of North Carolina. They document Moho holes, double Moho arrivals, and localized seismic scatters in the lithospheric mantle. One interpretation of these results is that portions of the over-thickened crust have delaminated (cf. Zandt et al., 2004), perhaps driving the uplift and rejuvenation of the southern Appalachian landscape. Further testing of this hypothesis is possible by continued collaborations between the geomorphic and geophysical communities and the arrival of the EarthScope USArray seismic observatory experiment to the eastern United States in 2013.
Conclusions
Our results show that topography in the Cullasaja River basin, and likely much of the west-draining southern Appalachians, is in a transient state of adjustment to a newly imposed regional base level (Gallen et al., 2011), and thus it is not in a dynamic equilibrium. Relief has increased here >150% since the Miocene, predating the amplification of glacial-interglacial cycles that initiated in the Pliocene and continue today. Our results favor the hypothesis that some form of dynamic mantle forcing has caused epeirogenic uplift of the Appalachians that began in the Miocene, because it can explain the generation of knickpoints and the preservation of a relict landscape in the Cullasaja basin that are difficult to account for in the context of climate change alone. Importantly, it appears that this event may be related to the increase in grain size and rate of sediments delivered to basins both east (Poag and Sevon, 1989; Pazzaglia and Brandon, 1996) and west (Potter, 1955; Galloway et al., 2011) of the Appalachian mountains, implying that the surface response to relief generation in the Cullasaja basin is likely related to a broad, regional phenomenon. This research sheds light on a long-standing enigma in the geosciences; yet, the results presented here also bring up new questions and testable hypotheses about the geomorphology and late Cenozoic geodynamic evolution of the southern Appalachians.
Acknowledgments
We are forever indebted to K.L. Frankel, who passed untimely before the completion of this study. The conception of this project would not have been possible without his interest and enthusiasm about the evolution of the Appalachians. We would like to thank Marin Clark and an anonymous reviewer for excellent reviews that helped improve and refine this manuscript. Chuck Harrington helped clarify an earlier version of this manuscript. Bernie Housen is credited with encouraging us to submit this work to GSA Today.
REFERENCES CITED
- Ahnert, F., 1970, Functional relationships between denudation, relief, and uplift in large, mid-latitude drainage basins: American Journal of Science, v. 268, no. 3, p. 243–263, doi: 10.2475/ajs.268.3.243.
- Berlin, M.M., and Anderson, R.S., 2007, Modeling of knickpoint retreat on the Roan Plateau, western Colorado: Journal of Geophysical Research, v. 112, F03S06, doi: 10.1029/2006jf000553.
- Bishop, P., 2007, Long-term landscape evolution: Linking tectonics and surface processes: Earth Surface Processes and Landforms, v. 32, no. 3, p. 329–365, doi: 10.1002/esp.1493.
- Boettcher, S.S., and Milliken, K.L., 1994, Mesozoic-Cenozoic unroofing of the southern Appalachian Basin: Apatite fission track evidence from Middle Pennsylvanian sandstones: The Journal of Geology, v. 102, no. 6, p. 655–668.
- Clark, M.K., Maheo, J.S., Saleeby, J., and Farley, K.A., 2005, The non-equilibrium landscape of the southern Sierra Nevada, California: GSA Today, v. 15, no. 9, p. 4–10, doi: 10:1130/1052-5173(2005)015<4:TNELOT>2.0.CO;2.
- Crosby, B.T., and Whipple, K.X., 2006, Knickpoint initiation and distribution within fluvial networks: 236 waterfalls in the Waipaoa River, North Island, New Zealand: Geomorphology, v. 82, no. 1–2, p. 16–38.
- Davis, W.M., 1889, The river and valleys of Pennsylvania: National Geographic Magazine, v. 1, p. 183–253.
- Flint, J.J., 1974, Stream gradient as a function of order, magnitude, and discharge: Water Resources Research, v. 10, no. 5, p. 969–973, doi: 10.1029/WR010i005p00969.
- Gallen, S.F., Wegmann, K.W., Frankel, K.L., Hughes, S., Lewis, R.Q., Lyons, N., Paris, P., Ross, K., Bauer, J.B., and Witt, A.C., 2011, Hillslope response to knickpoint migration in the Southern Appalachians: Implications for the evolution of post-orogenic landscapes: Earth Surface Processes and Landforms, v. 36, no. 9, p. 1254–1267, doi: 10.1002/esp.2150.
- Galloway, W.E., Whiteaker, T.L., and Ganey-Curry, P., 2011, History of Cenozoic North American drainage basin evolution, sediment yield, and accumulation in the Gulf of Mexico basin: Geosphere, v. 7, no. 4, p. 938–973, doi: 10.1130/ges00647.1.
- Grand, S.P., van de Hilst, R.D., and Widiyantoro, S., 1997, Global seismic tomography: A snapshot of convection in the earth: GSA Today, v. 7, no. 4, p. 1–7.
- Hack, J.T., 1960, Interpretation of erosional topography in humid temperate regions: American Journal of Science, v. 258-A, p. 80–97.
- Hack, J.T., 1982, Physiographic divisions and differential uplift in the Piedmont and Blue Ridge: U.S. Geological Survey Professional Paper 1265-P, p. 1–49.
- Hancock, G., and Kirwan, M., 2007, Summit erosion rates deduced from 10Be: Implications for relief production in the central Appalachians: Geology, v. 35, no. 1, p. 89–92, doi: 10.1130/g23147a.1.
- Hatcher, R.D., Jr., 1989, Tectonic synthesis of the U.S. Appalachians, in Hatcher, R.D., Jr., Thomas, W.A., and Viele, G.W., eds., The Appalachian-Ouachita Orogen in the United States: Boulder, Colorado, USA Geological Society of America Decade of North American Geology, v. F-2, p. 511–531.
- Karlstrom, K.E., Coblentz, D., Dueker, K., Ouimet, W., Kirby, E., Van Wijk, J., Schmandt, B., Kelley, S., Lazear, G., Crossey, L.J., Crow, R., Aslan, A., Darling, A., Aster, R., MacCarthy, J., Hansen, S.M., Stachnik, J., Stockli, D.F., Garcia, R.V., Hoffman, M., McKeon, R., Feldman, J., Heizler, M., Donahue, M.S., and the CREST Working Group, 2012, Mantle-driven dynamic uplift of the Rocky Mountains and Colorado Plateau and its surface response: Toward a unified hypothesis: Lithosphere, v. 4, no. 1, p. 3–22, doi: 10.1130/L150.1.
- Korup, O., 2006, Rock-slope failure and the river long profile: Geology, v. 34, no. 1, p. 45–48, doi: 10.1130/G21959.1.
- Loget, N., and Van Den Driessche, J., 2009, Wave train model for knickpoint migration: Geomorphology, v. 106, no. 3–4, p. 376–382.
- Matmon, A., Bierman, P.R., Larsen, J., Southworth, S., Pavich, M., and Caffee, M., 2003, Temporally and spatially uniform rates of erosion in the southern Appalachian Great Smoky Mountains: Geology, v. 31, no. 2, p. 155–158, doi: 10.1130/0091-7613(2003)031<0155:TASURO>2.0.co;2.
- Mills, H.H., 2000, Apparent increasing rates of stream incision in the eastern United States during the late Cenozoic: Geology, v. 28, no. 10, p. 955–957, doi: 10.1130/0091-7613(2000)28<955:AIROSI>2.0.co;2.
- Molnar, P., 2004, Late Cenozoic increase in accumulation rates of terrestrial sediment: How might climate change have affected erosion rates?: Annual Review of Earth and Planetary Sciences, v. 32, no. 1, p. 67–89, doi: 10.1146/annurev.earth.32.091003.143456.
- Montgomery, D.R., and Brandon, M.T., 2002, Topographic controls on erosion rates in tectonically active mountain ranges: Earth and Planetary Science Letters, v. 201, no. 3–4, p. 481–489.
- Montgomery, D.R., and Foufoula-Georgiou, E., 1993, Channel network source representation using digital elevation models: Water Resources Research, v. 29, no. 12, p. 3925–3934.
- Niemann, J.D., Gasparini, N.M., Tucker, G.E., and Bras, R.L., 2001, A quantitative evaluation of Playfair’s law and its use in testing long-term stream erosion models: Earth Surface Processes and Landforms, v. 26, no. 12, p. 1317–1332, doi: 10.1002/esp.272.
- Norton, K.P., von Blanckenburg, F., Schlunegger, F., Schwab, M., and Kubik, P.W., 2008, Cosmogenic nuclide-based investigation of spatial erosion and hillslope channel coupling in the transient foreland of the Swiss Alps: Geomorphology, v. 95, no. 3–4, p. 474–486.
- Pazzaglia, F.J., and Brandon, M.T., 1996, Macrogeomorphic evolution of the post-Triassic Appalachian mountains determined by deconvolution of the offshore basin sedimentary record: Basin Research, v. 8, no. 3, p. 255–278, doi: 10.1046/j.1365-2117.1996.00274.x.
- Peizhen, Z., Molnar, P., and Downs, W.R., 2001, Increased sedimentation rates and grain sizes 2–4 myr ago due to the influence of climate change on erosion rates: Nature, v. 410, no. 6831, p. 891–897.
- Poag, C.W., and Sevon, W.D., 1989, A record of Appalachian denudation in post-rift Mesozoic and Cenozoic sedimentary deposits of the U.S. Middle Atlantic continental margin: Geomorphology, v. 2, no. 1–3, p. 119–157.
- Potter, P.E., 1955, The petrology and origin of the Lafayette gravel part 2. Geomorphic history: The Journal of Geology, v. 63, no. 2, p. 115–132.
- Prince, P.S., Spotila, J.A., and Henika, W.S., 2011, Stream capture as driver of transient landscape evolution in a tectonically quiescent setting: Geology, v. 39, no. 9, p. 823–826, doi: 10.1130/G32008.1.
- Ren, Y., Stutzmann, E., van der Hilst, R.D., and Besse, J., 2007, Understanding seismic heterogeneities in the lower mantle beneath the Americas from seismic tomography and plate tectonic history: Journal of Geophysical Research, v. 112, B01302, doi: 10.1029/2005jb004154.
- Reusser, L.J., Bierman, P.R., Pavich, M.J., Zen, E., Larsen, J., and Finkel, R., 2004, Rapid Late Pleistocene incision of Atlantic passive-margin river gorges: Science, v. 305, 5683, p. 499–502, doi: 10.1126/science.1097780.
- Reusser, L.J., Bierman, P.R., Pavich, M.J., Larsen, J., and Finkel, R., 2006, An episode of rapid bedrock channel incision during the last glacial cycle, measured with 10Be: American Journal of Science, v. 306, no. 2, p. 69–102.
- Schumm, S.A., 1993, River response to base-level change; implications for sequence stratigraphy: The Journal of Geology, v. 101, no. 2, p. 279–294.
- Sella, G.F., Stein, S., Dixon, T.H., Craymer, M., James, T.S., Mazzotti, S., and Dokka, R.K., 2007, Observation of glacial isostatic adjustment in “stable” North America with GPS: Geophysical Research Letters, v. 34, L02306, doi: 10.1029/2006gl027081.
- Thelin, G.P., and Pike, R.J., 1991, Landforms of the conterminous United States—A digital shaded-relief portrayal: USGS Map 2206, scale 1:3,500,000, 1 sheet.
- Thomas, D.J., 1996, Soil survey of Macon County, North Carolina: Washington D.C., U.S. Department of Agriculture Natural Resources Conservation Service, 322 p.
- Wagner, L.S., Stewart, K., and Metcalf, K., 2012, Crustal-scale shortening structures beneath the Blue Ridge Mountains, North Carolina, USA: Lithosphere, v. 4, no. 3, p. 242–256, doi: 10.1130/L184.1.
- Whipple, K.X., and Tucker, G.E., 1999, Dynamics of the stream-power river incision model: Implications for height limits of mountain ranges, landscape response timescales, and research needs: Journal of Geophysical Research, v. 104, B8, p. 17,661–17,674, doi: 10.1029/1999jb900120.
- Wobus, C., Whipple, K.X., Kirby, E., Snyder, N., Johnson, J., Spyropolou, K., Crosby, B., and Sheehan, D., 2006, Tectonics from topography: Procedures, promise, and pitfalls, in Willett, S.D., Hovius, N., Brandon, M.T., and Fisher, D.M., eds., Tectonics, Climate and Landscape Evolution: Geological Society of America Special Papers 398, p. 55–74, doi: 10.1130/2006.2398(04).
- Wobus, C.W., Tucker, G.E., and Anderson, R.S., 2010, Does climate change create distinctive patterns of landscape incision?: Journal of Geophysical Research, v. 115, F04008, doi: 10.1029/2009jf001562.
- Wooten, R.M., Gillon, K.A., Witt, A.C., Latham, R.S., Douglas, T.J., Bauer, J.B., Fuemmeler, S.J., and Lee, L.G., 2008, Geologic, geomorphic, and meteorological aspects of debris flows triggered by Hurricanes Frances and Ivan during September 2004 in the Southern Appalachian Mountains of Macon County, North Carolina (southeastern USA): Landslides, v. 5, no. 1, p. 31–44, doi: 10.1007/s10346-007-0109-9.
- Wu, X., Heflin, M.B., Schotman, H., Vermeersen, B.L.A., Dong, D., Gross, R.S., Ivins, E.R., Moore, A.W., and Owen, S.E., 2010, Simultaneous estimation of global present-day water transport and glacial isostatic adjustment: Nature Geoscience, v. 3, no. 9, p. 642–646.
- Zandt, G., Gilbert, H., Owens, T.J., Ducea, M., Saleeby, J., and Jones, C.H., 2004, Active foundering of a continental arc root beneath the southern Sierra Nevada in California: Nature, v. 431, 7004, p. 41–46.
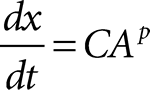
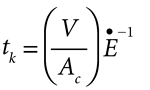