Full Text View
Volume 28 Issue 10 (October 2018)
GSA Today
Article, pp. 4-10 | Abstract | PDF (1MB)
The other biodiversity record: Innovations in animal-substrate interactions through geologic time
Cover Image
Table of Contents
- Introduction
- Concepts and Methods
- Results
- Discussion: Significance, Caveats, and Prospects
- Acknowledgments
- References Cited
Search GoogleScholar for
Search GSA Today

Abstract
Tracking biodiversity changes based on body fossils through geologic time became one of the main objectives of paleontology in the 1980s. Trace fossils represent an alternative record to evaluate secular changes in diversity. A quantitative ichnologic analysis, based on a comprehensive and global data set, has been undertaken in order to evaluate temporal trends in diversity of bioturbation and bioerosion structures. The results of this study indicate that the three main marine evolutionary radiations (Cambrian Explosion, Great Ordovician Biodiversification Event, and Mesozoic Marine Revolution) detected in the body-fossil record are also expressed in the trace-fossil record. Analysis of ichnodiversity trajectories in marine environments supports Sepkoski’s logistic model, which was originally based on analysis of marine body fossils. The trace-fossil record of continental environments suggests variable rates of increases in ichnodiversity, with major radiations in the Ludlow–Early Devonian, Cisuralian, Early Jurassic, Late Cretaceous, and Eocene, and slower increases or plateaus in between these periods. Our study indicates that ichnologic information represents an independent line of evidence that yields valuable insights to evaluate paleobiologic megatrends.
Manuscript received 17 Dec. 2017. Revised manuscript received 4 July 2018. Manuscript accepted 18 July 2018. Posted 25 September 2018.
© The Geological Society of America, 2018. CC-BY-NC.
Introduction
The astounding diversity of animals in modern oceans and continents is the result of macroevolutionary processes operating in deep time. Conservative estimates suggest the modern biosphere hosts close to 13 million species, of which approximately only 4% are marine (Benton, 2001). Despite the impressive growth of fields exploring other sources of data (e.g., molecular), the fossil record is arguably still the main line of evidence to reconstruct the diversity of life through time (Valentine, 1969; Raup, 1972, 1979; Bambach, 1977; Sepkoski, 1978, 1979, 1984, 1997). However, this has been marked by controversies regarding the nature of diversity trajectories and their potential biases (e.g., Sepkoski et al., 1981; Alroy, 2010; Crampton et al., 2003; Holland, 2010; Bush and Bambach, 2015). In these studies, diversity has been invariably assessed based on body fossils.
Trace fossils represent an alternative record to assess secular changes in biodiversity. Trace-fossil data were given less attention and were considered briefly in only one of the more classic studies of biodiversity through time (e.g., the so-called consensus paper by Sepkoski et al., 1981; see also Miller, 2009). Ichnologic information in the consensus paper was based on early attempts of quantifying trace-fossil diversity, which were supported by the very limited data available at the time (Seilacher, 1974, 1977). The decision by Dolf Seilacher to decline co-authorship of the consensus paper reflects his doubts with respect to the support that trace-fossil evidence was actually providing to the diversity curves based on body fossils. In a letter addressed to Sepkoski, dated 23 February 1981, Seilacher stated, “By lumping the two groups you may get a curve that pleases you, but this might be an accident. The curve you have in mind (based on the record of body fossils) is mainly one of shallow marine diversity. Including the flysch (i.e., the deepwater) counts (which by their high diversity influence the results very much), I am afraid will do no justice to the cause, although the result may fit the general picture” (Miller, 2009, p. 379).
No attempts to produce global comprehensive compilations have been done since the pioneer work by Seilacher (see also Crimes, 1974). Subsequent ichnologic compilations focused on specific environments (e.g., Buatois et al., 1998; Orr, 2001; Uchman, 2004; Minter et al., 2016a, 2016b, 2017) or evolutionary radiations (e.g., Mángano and Droser, 2004; Mángano and Buatois, 2014; Buatois et al., 2016a), rather than on the whole Phanerozoic. In this study we tackle this issue based on a systematic and global compilation of trace-fossil data in the stratigraphic record. We show that quantitative ichnologic analysis indicates that the three main marine evolutionary radiations inferred from body fossils, namely the Cambrian Explosion, Great Ordovician Biodiversification Event, and Mesozoic Marine Revolution, are also expressed in the trace-fossil record. In addition, the trace- fossil record of continental environments suggests variable rates of increases in diversity, with major radiations in the Ludlow–Early Devonian, Cisuralian, Early Jurassic, Late Cretaceous, and Eocene, and slower increases or plateaus in between these periods.
--------
2 GSA Data Repository Item 2018307, Fig. DR1, Tables DR1–DR3, and further comments on concepts and methods, is available online at www.geosociety.org/datarepository/2018/.
Concepts and Methods
Trace fossils comprise distinctive structures of biogenic origin, reflecting organism behaviors while interacting with the substrate (Fig. 1). Trace fossils may be preserved in a wide variety of substrates. Bioturbation structures occur in sediment, whereas bioerosion structures are produced in rigid substrates, such as hardgrounds, clasts, bones, or rocks (Frey and Wheatcroft, 1989). Although the same type of trace-fossil can be produced by phylogenetically unrelated animals, in many instances trace fossils can be attributed to a producer with variable levels of confidence. For example, the branching burrow system Ophiomorpha is commonly regarded as produced by decapod crustaceans, typically callianassids (Fig. 1) (Frey et al., 1978). However, such links are not possible to establish in the case of ichnofossils having less distinct morphologic features. The trace-fossil record encompasses marine and continental environments and spans from Ediacaran to Holocene (Buatois and Mángano, 2016).
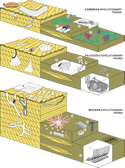
Ichnologic equivalents of Sepkoski’s evolutionary faunas. Representative elements of the Cambrian evolutionary fauna include trilobite trace fossils, such as Rusophycus (Ru), Cruziana (Cr) and Dimorphichnus (Di), and the inarticulate brachiopod burrow Lingulichnus (Li). Trace fossils of worm-like organisms include Syringomorpha (Sy) and Oldhamia (Ol), whereas Climactichnites (Cl) has been attributed to mollusk-like producers. Examples of the Paleozoic evolutionary fauna include ichnotaxa produced by worm-like animals, such as Daedalus (Da), Arthrophycus (Ar), Heimdallia (He), and Dictyodora (Dy). The Modern evolutionary fauna is dominated by crustacean burrows, such as Psilonichnus (Ps), Ophiomorpha (Op), Thalassinoides (Th), and Sinusichnus (Si), as well as bivalves, suchas Hillichnus (Hi), and irregular echinoids, such as Scolicia (Sc). Structures produced by worm-like organisms include Lapispira (La), Schaubcylindrichnus (Sch), Phoebichnus (Ph), and Haentzschelinia (Ha). For bibliographic sources, see supplementary material in the GSA Data Repository (see text footnote 1).
Whereas the main focus of studies on biodiversity has been on the number of species or higher taxa (Sepkoski, 1997), ichnodiversity (or trace-fossil diversity) studies focus on the different behaviors involved in animal-substrate interactions. Ichnodiversity refers to the number of trace-fossil types (ichnotaxa) present in any given assemblage or geologic period, therefore providing a measurement of behavioral richness (Buatois and Mángano, 2011, 2013). Ichnogeneric compilation was based on literature and personal data (GSA Data Repository Tables DR1 and DR21). The total number of invertebrate ichnogenera identified as valid is 534, encompassing 428 for bioturbation structures and 106 for bioerosion structures (updated from Buatois et al., 2017). We follow the common practice of assessing ichnodiversity at ichnogeneric rank, because there is a general agreement that taxonomy is more firmly established at ichnogenus level than at ichnospecies rank. Only invertebrate trace-fossil data were considered. Individual curves were produced for continental, shallow-marine, and deep-marine bioturbation, and marine and continental bioerosion (Fig. 2). Additional diversity curves were compiled for all marine bioturbation, all marine (bioerosion plus bioturbation), and all continental (bioerosion plus bioturbation) ichnogenera. A rarefaction analysis of the data pertaining to the Cambrian and Ordovician portions of the marine curves showing rapid ichnodiversity increases was undertaken to evaluate the effects of sample size on the curves (Buatois et al., 2016a). Diversity data for the continental Paleozoic were standardized by using the residuals method to evaluate potential sampling biases regarding the abundance of nonmarine clastic rock volume, the number of ichnofossil-bearing formations, and the number of trace-fossil assemblages documented (Minter et al., 2017). Potential biases are discussed in the supplementary material (see footnote 1).
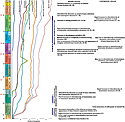
Ichnodiversity changes and milestones in animal-substrate interactions through geologic time as reflected by the trace-fossil record. Arrows indicate mass extinctions. Numbers correspond to bibliographic sources indicated in the supplementary material in the GSA Data Repository (see text footnote 1).
Results
Behavioral innovations seem to have taken place in pulses rather than at a steady pace (Fig. 2). For marine environments, a 433% increase in ichnodiversity occurred during the Terraneuvian, a 48% increase took place between the Early and Late Ordovician, and a more protracted and modest increase occurred later in the Mesozoic with increases in the Early Jurassic (8%) and Late Cretaceous (20%), totaling an overall increase of 37% (between the Late Triassic and Late Cretaceous) (Table DR3 [see footnote 1]).
The Terraneuvian increase, attributed to the Cambrian Explosion, is restricted to bioturbation (Mángano and Buatois, 2014; Buatois et al., 2016a). The Cambrian Explosion is characterized by the rise of the Cambrian evolutionary fauna, which was dominated by trilobites, one of the most significant elements not only based on the body-fossil record, but also from an ichnologic perspective (Seilacher, 1985). In addition, inarticulate brachiopods, another important component, are represented by the ichnogenus Lingulichnus (Pemberton and Kobluk, 1978). However, the high ichnodiversity that characterizes the Cambrian reflects the activity of soft-bodied clades that are poorly known from the body-fossil record (and, therefore, not listed as members of the Cambrian evolutionary fauna). Several ichnogenera are restricted to the Cambrian (e.g., Climactichnites, Oldhamia, Syringomorpha). Trilobite trace fossils, as well as those ichnogenera showing stratigraphic ranges restricted to the Cambrian, may be regarded as the ichnologic equivalent of Sepkoski’s Cambrian evolutionary fauna (Figs. 1 and DR1 [see footnote 1]).
The Ordovician increase in ichnodiversity is attributed to the Great Ordovician Biodiversification Event and is reflected not only by an increase in diversity of bioturbation, but also by bioerosion structures (Ordovician bioerosion revolution of Wilson and Palmer, 2006) (Fig. 2, Table DR3 [see footnote 1]). This revolution in the ability of organisms to penetrate skeletal material and hardgrounds occurred ~80 m.y. after the Cambrian Explosion in bioturbation (Buatois et al., 2016a). The Great Ordovician Biodiversification Event signals a shift from dominance of elements of the Cambrian evolutionary fauna to those of the Paleozoic and Modern evolutionary faunas (Sepkoski, 1981; Miller and Connolly, 2001). Articulate brachiopods, rugose and tabulate corals, and crinoids are dominant elements of the Paleozoic evolutionary fauna (Sepkoski, 1981). Unsurprisingly given their modes of life, none of these clades are significant from an ichnologic standpoint, obscuring characterization of an equivalent of the Paleozoic evolutionary fauna. However, a few ichnogenera showing restriction or peak abundance during the Paleozoic (e.g., Arthrophycus, Dictyodora, Daedalus, Heimdallia) may be regarded as typical of the Paleozoic evolutionary fauna (Figs. 1 and DR1 [see footnote 1]). In addition, the trace-fossil record of the Great Ordovician Biodiversification Event reflects the dilution of trilobite faunas inferred from the body-fossil record. Contribution of trilobite-produced trace fossils to alpha ichnodiversity at ichnospecies level in the Tremadocian averages 41.5%, whereas only a 30.6% was attained in the Floian–Darriwilian, and just 12.1% for the Sandbian–Hirnantian (Mángano et al., 2016).
The Mesozoic ichnodiversity increase (Fig. 2, Table DR3 [see footnote 1]), concomitant with the rise to dominance of the modern evolutionary fauna (MEF), is attributed to the Mesozoic Marine Revolution. Bivalves, gastropods, echinoids, crustaceans, and marine vertebrates are dominant elements (Sepkoski, 1981). Irregular echinoids, crustaceans, and bivalves are the most important bioturbators in marine settings, in addition to worms (Buatois et al., 2016b), illustrating the ichnologic equivalent of the MEF (Figs. 1 and DR1 [see footnote 1]). Heart urchins (spatangoids), known since the Early Jurassic, are the producers of the ichnogenera Bichordites, Cardioichnus, and Scolicia (Smith and Crimes, 1983; Plaziat and Mahmoudi, 1988), all typical bioturbators in post-Paleozoic seas. Crustacean burrows include ichnogenera that occurred for the first time during the Mesozoic (e.g., Ophiomorpha, Psilonichnus, Sinusichnus, Spongeliomorpha) and a few that are known since the Paleozoic but became more abundant as a result of the Mesozoic Marine Revolution (Gyrolithes, Thalassinoides) (Carmona et al., 2004). Other crustacean ichnotaxa occurred for the first time in the Neogene (Parmaichnus, Lepeichnus). The most common trace fossils produced by bivalves (Protovirgularia, Lockeia), although known since the early Paleozoic, became more abundant since the Mesozoic (Buatois et al., 2016b). Siphonichnus is common in the late Paleozoic, reflecting an evolutionary radiation of infaunal bivalves, but it is particularly abundant since the Triassic (Knaust, 2015). Hillichnus, attributed to the activities of tellinacean bivalves (Bromley et al., 2003), is a product of the Mesozoic Marine Revolution. Some of the trace fossils produced by worms (e.g., Haentzschelinia, Lapispira, Patagonichnus, Phoebichnus) are only known in post-Paleozoic marine strata, but the vast majority (e.g., Cylindrichnus, Macaronichnus, Schaubcylindrichnus) have been documented since the Paleozoic. However, some of these ichnogenera became much more common since the Mesozoic (Buatois et al., 2016b). The most significant bioeroders were sponges, gastropods, bivalves, echinoids, and worms. The sponge bioerosion ichnogenus Entobia is known since the Devonian (Tapanila, 2006), but it became abundant in the Mesozoic, when various ichnospecies originated. Significant bioerosion innovations are represented by Radulichnus (gastropods and chitons), Teredolites (pholadid bivalves), Gnathichnus (regular echinoids), and Maeandropolydora (spionid polychaetes) (Taylor and Wilson, 2003; Radley, 2010; Villegas-Martín et al., 2012).
Ichnodiversity in continental environments (Fig. 2, Table DR3 [see footnote 1]) is more difficult to evaluate due to the patchiness of the trace-fossil record (Minter et al., 2016a, 2016b, 2017). A 967% ichnodiversity increase characterizes the Silurian–Devonian transition, followed by a plateau until the Early Mississippian (Table DR3), although the latter most likely reflects the scarcity of Upper Devonian continental strata (Minter et al., 2016b). New rises in ichnodiversity took place during the Cisuralian (65%) and the Early Jurassic (22%) (Table DR3). Subsequent to this peak, ichnodiversity experienced a gradual and continuous increase. The earliest uncontroversial evidence of continental bioerosion is from the late Permian (Labandeira et al., 2017), but it is by the Late Triassic when rapid diversification took place (Tapanila and Roberts, 2012). The earliest record of bone bioerosion is from the Early Jurassic (Xing et al., 2015). An increase in ichnodiversity of continental bioerosion occurred in the Late Cretaceous (100%) followed by a plateau that continues until the Holocene (Fig. 2, Table DR3). Overall, the ichnodiversity increases that took place in continental environments by the end of the Mesozoic may reflect the appearance of sophisticated insect nesting structures in soils (Genise, 2016).
Discussion: Significance, Caveats, and Prospects
The concepts of Cambrian Explosion, Great Ordovician Biodiversification Event, and Mesozoic Marine Revolution are all based on body fossils. The fact that these evolutionary radiations are also reflected by the trace-fossil record provides independent support to the notion that these were truly macroevolutionary events, rather than taphonomic artifacts (Buatois and Mángano, 2016). Although some hard-bodied invertebrates (e.g., bivalves) produce trace fossils, most biogenic structures are made by soft-bodied organisms (sometimes collectively and informally referred to as worms, but in fact encompassing a wide variety of clades) or poorly skeletonized invertebrates (e.g., thalassinidean crustaceans). The trace-fossil record provides information on animals that are typically underrepresented in the body-fossil record. Assessing the record of small and soft-bodied organisms making the majority of the biomass (“tackling the 99%” in Sperling’s 2013 analogy) is of fundamental importance to reconstructing the history of life. Also, our results are consistent with the observation that each evolutionary fauna shows more ecologic complexity than the previous one, revealing an increased use of the ecospace (Bambach et al., 2007; Bush et al., 2007).
Diversification curves may adopt three different patterns, as illustrated by the straight (representing additive increase), exponential (implying doubling of diversity within fixed units of time if speciation and extinction rates remain constant), and logistic (comprising initial slow diversification followed by a rapid rise and a plateau) models (Benton, 2001). In turn, logistic models represent equilibrium models because they imply that global equilibria in diversity is attained, whereas exponential models are non-equilibrium models implying that a ceiling to diversity has not been reached or that there is no such ceiling (Benton, 2001). Diversification of marine invertebrates is apparently best described by a logistic model (Sepkoski, 1978, 1984), but diversification of continental biotas may have followed an exponential model (Benton, 1985, 2001; Labandeira and Sepkoski, 1993). However, in recent years it has been argued that fluctuating equilibrium diversities through time may produce a pattern of intervals of relative stability alternating with times of radiations, which is apparently similar to a coupled logistic model, but resulting from a different underlying evolutionary dynamics (Alroy, 2010; Foote, 2010; Bush et al., 2016).
According to the logistic model, marine evolutionary radiations display the pattern of early slow growth, subsequent rapid growth, and final slowing of growth reaching a plateau (Sepkoski, 1978, 1984). This is particularly illustrated by the Cambrian Explosion and the Great Ordovician Biodiversification Event, which displayed very rapid growths of diversity in their initial stages until reaching a plateau (Sepkoski, 1978, 1979). In contrast, diversity rose more slowly during the initial phase of the Mesozoic Marine Revolution and apparently has not reached a plateau yet (Sepkoski, 1981, 1984). The three-phase kinetic model developed by Sepkoski (1984) provided an analytical view of marine diversification with different global equilibria for each of the three evolutionary radiations (but see Kowalewski et al., 2006; Kiessling et al., 2008; Alroy et al., 2008; Alroy, 2010, 2014). Studies based on standardized curves indicated that late Cenozoic diversity is only slightly higher than the Paleozoic maximum (e.g., Alroy et al., 2008; Alroy, 2010, 2014). However, recent work shows a pattern that is more consistent with the original Sepkoski’s curves, indicating Cenozoic diversity levels that doubled Paleozoic values (Bush and Bambach, 2015).
The curves for marine invertebrate trace fossils show a similar trajectory to that of body fossils (Sepkoski, 1997), providing empiric independent support to the three-phase kinetic (logistic) model (Sepkoski, 1984) and indicating similar diversity trajectories for animal diversity and their behaviors. The explosive Terraneuvian diversification in bioturbation was preceded by a slow increase during the terminal Ediacaran and followed by a plateau during the middle to late Cambrian, underscoring the logistic nature of the Cambrian Explosion. This diversification preceded the Cambrian Explosion as indicated by body fossils, which took place later in the early Cambrian (Mángano and Buatois, 2014). A similar case of logistic pattern of diversification can be made for the Great Ordovician Biodiversification Event with a rapid rise taking place through the Ordovician and a plateau lasting for roughly the remaining of the Paleozoic. In turn, the rapid rise in ichnodiversity of the Late Cretaceous was preceded by a more protracted increase that started during the recovery after the end-Permian mass extinction and was followed by a plateau until the present. This represents a departure from the diversity trajectory of post-Paleozoic faunas, which does not show any evidence of a plateau (Sepkoski, 1981, 1984).
Variable rates of increases in ichnodiversity are detected for continental bioturbation, with major radiations in the Ludlow–Early Devonian, Cisuralian, Early Jurassic, Late Cretaceous, Eocene, and Miocene (Fig. 2, Table DR3 [see footnote 1]). No plateau indicative of an equilibrium stage is apparent, suggesting that the invasion of the land is still an ongoing process (Miller and Labandeira, 2002). This is consistent with the non-equilibrium nature of models currently envisaged for diversification in continental environments based on body fossils (Benton, 2001). On the contrary, a plateau seems to have been reached by the end of the Mesozoic in the case of continental bioerosion. However, our knowledge of continental bioerosion lags considerably behind that of bioturbation, so available patterns should be taken with extreme caution.
Forty years of ichnologic research since the compilation by Dolf Seilacher prompt us to question if his reluctance to get involved in the consensus papers is still justified by the now available evidence. Our study shows that after the initial burst in ichnodiversity that took place during the Cambrian Explosion, subsequent diversification was most clearly manifested in the deep sea (83% increase between the end of the Cambrian and the end of the Ordovician and 58% between the Middle Jurassic and the Eocene) (Table DR3 [see footnote 1]). However, the increase was not restricted to the deep sea, but also took place in shallow water (42% increase between the end of the Cambrian and the end of the Ordovician and 26% through the whole Mesozoic) (Table DR3). Further ichnodiversity increases are apparent for bioerosion, which cannot be attributed to innovations in the deep sea (Fig. 2).
However, Seilacher’s original compilations, showing constant ichnodiversity levels in shallow water and increased diversification in the deep sea, were not of global ichnodiversity, but of maximum alpha ichnodiversity. Therefore, these curves reflect richness at a community level rather than globally. Ongoing work is attempting to produce a database compiling alpha ichnodiversity for specific environments through the Phanerozoic in order to evaluate changes in within- and inter-habitat ichnodiversity. Previous work on deep-sea trace fossils by Uchman (2004) suggested a more nuanced scenario to that originally indicated by Seilacher (1974, 1977). Although an overall increase in maximum alpha ichnodiversity is apparent, this trend has been punctuated by several fluctuations, including significantly low levels from the Carboniferous to the Middle Jurassic (Uchman, 2004). Comparative analysis of brackish-water marginal-marine environments indicates that, even in these stressful settings, alpha ichnodiversity has increased through the Phanerozoic (Buatois et al., 2005).
The explosive development of analytical paleobiology demonstrates the need for standardizing trace-fossil data in the same fashion that has been done with body-fossil data. Ongoing ichnologic work is showing the usefulness of this approach, which has produced robust trace-fossil data to compare the Cambrian Explosion and the Great Ordovician Biodiversification Event (Buatois et al., 2016a), and to reconstruct the Paleozoic invasion of the continents (Minter et al., 2017). In conclusion, ichnologic information has a lot to offer to the reconstruction of the history of life, representing an independent line of evidence that yields valuable insights to evaluate paleobiologic megatrends.
Acknowledgments
A number of colleagues provided valuable feedback, particularly Nic Minter (standardized continental ichnodiversity data), Ricardo Olea (rarefaction analysis for marine ichnodiversity data), Mark Wilson (macrobioerosion), and Max Wisshak (microbioerosion). We are very thankful to Kai Zhou who assisted us with plotting the information in tables and charts. Doug Erwin, two anonymous reviewers, and editor Jerry Dickens provided highly useful comments that considerably improved the paper.
References Cited
- Alroy, J., 2010, The shifting balance of diversity among major marine animal groups: Science, v. 329, p. 1191–1194, https://doi.org/10.1126/ science.1189910.
- Alroy, J., 2014, Accurate and precise estimates of origination and extinction rates: Paleobiology, v. 40, p. 374–397, https://doi.org/10.1666/13036.
- Alroy, J., Aberhan, M., Bottjer, D.J., Foote, M., Fürsich, F.T., Harries, P.J., Hendy, A.J.W., Holland, S.M., Ivany, L.C., Kiessling, W., Kosnik, M.A., Marshall, C.R., McGowan, A.J., Miller, A.I., Olszewski, T.D., Patzkowsky, M.E., Peters, S.E., Villier, L., Wagner, P.J., Bonuso, N., Borkow, P.S., Brenneis, B., Clapham, M.E., Fall, L.M., Ferguson, C.A., Hanson, V.L., Krug, A.Z., Layou, K.M., Leckey, E.H., Nürnberg, S., Powers, C.M., Sessa, J.A., Simpson, C., Tomaovch, A., and Visaggi, C.C., 2008, Phanerozoic trends in the global diversity of marine invertebrates: Science, v. 321, p. 97–100, https://doi.org/10.1126/science. 1156963.
- Bambach, R.K., 1977, Species richness in marine benthic habitats through the Phanerozoic: Paleobiology, v. 3, p. 152–167, https://doi.org/10.1017/S0094837300005236.
- Bambach, R.K., Erwin, D.H., and Bush, A.M., 2007, Autecology and the filling of ecospace: Key metazoan radiations: Palaeontology, v. 50, p. 1–22, https://doi.org/10.1111/j.1475-4983.2006.00611.x.
- Benton, M.J., 1985, Patterns in the diversification of Mesozoic nonmarine tetrapods and problems in historical diversity analysis: Special Papers in Palaeontology, v. 33, p. 185–202.
- Benton, M.J., 2001, Biodiversity on land and in the sea: Geological Journal, v. 36, p. 211–230, https://doi.org/10.1002/gj.877.
- Bromley, R.G., Uchman, A., Gregory, M.R., and Martin, A.J., 2003, Hillichnus lobosensis igen. et isp. nov., a complex trace fossil produced by tellinacean bivalves, Paleocene, Monterey, California, USA: Palaeogeography, Palaeoclimatology, Palaeoecology, v. 192, p. 157–186, https://doi.org/10.1016/S0031-0182(02)00684-3.
- Buatois, L.A., and Mángano, M.G., 2011, Ichnology: Organism-substrate interactions in space and time: Cambridge, Cambridge University Press, 358 p., https://doi.org/10.1017/CBO9780511975622.
- Buatois, L.A., and Mángano, M.G., 2013, Ichnodiversity and ichnodisparity: Significance and caveats: Lethaia, v. 46, p. 281–292, https://doi.org/10.1111/let.12018.
- Buatois, L.A., and Mángano, M.G., 2016, Recurrent patterns and processes: The significance of ichnology in evolutionary paleoecology, in Mángano, M.G., and Buatois, L.A., eds., The trace-fossil record of major evolutionary changes, vol. 2: Mesozoic and Cenozoic: Amsterdam, Springer, Topics in Geobiology, v. 40, p. 449–473.
- Buatois, L.A., Mángano, M.G., Genise, J.F., and Taylor, T.N., 1998, The ichnologic record of the continental invertebrate invasion; evolutionary trends in environmental expansion, ecospace utilization, and behavioral complexity: Palaios, v. 13, p. 217–240, https://doi.org/10.2307/3515447.
- Buatois, L.A., Gingras, M.K., MacEachern, J., Mángano, M.G., Zonneveld, J.P., Pemberton, S.G., Netto, R.G., and Martin, A.J., 2005, Colonization of brackish-water systems through time: Evidence from the trace-fossil record: Palaios, v. 20, p. 321–347, https://doi.org/10.2110/palo.2004.p04-32.
- Buatois, L.A., Mángano, M.G., Olea, R.A., and Wilson, M.A., 2016a, Decoupled evolution of soft and hard substrate communities during the Cambrian Explosion and Great Ordovician Biodiversification Event: Proceedings of the National Academy of Sciences of the United States of America, v. 113, p. 6945–6948, https://doi.org/10.1073pnas. 1523087113.
- Buatois, L.A., Carmona, N.B., Curran, A.H., Netto, R.G., Mángano, M.G., and Wetzel, A., 2016b, The Mesozoic marine revolution, in Mángano, M.G., and Buatois, L.A., eds., The trace-fossil record of major evolutionary changes, vol. 2: Mesozoic and Cenozoic: Amsterdam, Springer, Topics in Geobiology, v. 40, p. 19–177.
- Buatois, L.A., Wisshak, M., Wilson, M.A., and Mángano, M.G., 2017, Categories of architectural designs in trace fossils: A measure of ichnodisparity: Earth-Science Reviews, v. 164, p. 102–181, https://doi.org/10.1016/j.earscirev.2016.08.009.
- Bush, A.M., and Bambach, R.K., 2015, Sustained Mesozoic–Cenozoic diversification of marine Metazoa: A consistent signal from the fossil record: Geology, v. 43, p. 979–982, https://doi.org/10.1130/G37162.1.
- Bush, A.M., Bambach, R.K., and Daley, G.M., 2007, Changes in theoretical ecospace utilization in marine fossil assemblages between the mid- Paleozoic and late Cenozoic: Paleobiology, v. 33, p. 76–97, https://doi.org/10.1666/06013.1.
- Bush, A.M., Hunt, G., and Bambach, R.K., 2016, Sex and the shifting biodiversity dynamics of marine animals in deep time: Proceedings of the National Academy of Sciences of the United States of America, v. 113, p. 14,073–14,078, https://doi.org/10.1073/pnas.1610726113.
- Carmona, N.B., Buatois, L.A., and Mángano, M.G., 2004, The trace fossil record of burrowing decapods crustaceans: Evaluating evolutionary radiations and behavioural convergence, in Webby, B.D., Mángano, M.G., and Buatois, L.A., eds., Trace fossils in evolutionary palaeoecology: Fossils and Strata, v. 51, p. 141–153.
- Crampton, J.S., Beu, A.G., Cooper, R.A., Jones, C.M., Marshall, B., and Maxwell, P.A., 2003, Estimating the rock volume bias in paleobiodiversity studies: Science, v. 301, p. 358–360, https://doi .org/10.1126/science.1085075.
- Crimes, T.P., 1974, Colonisation of the early ocean floor: Nature, v. 248, p. 328–330, https://doi.org/10.1038/248328a0.
- Foote, M., 2010, The geological history of biodiversity, in Bell, M.A., Futuyma, D.J., Eanes, W.F., and Levinton, J.S., eds., Evolution Since Darwin: The First 150 Years: Sunderland, Massachusetts, Sinauer, p. 479–510.
- Frey, R.W., and Wheatcroft, R.A., 1989, Organism-substrate relations and their impact on sedimentary petrology: Journal of Geological Education, v. 37, p. 261–279, https://doi.org/10.5408/00 22-1368-37.4.261.
- Frey, R.W., Howard, J.D., and Pryor, W.A., 1978, Ophiomorpha: Its morphologic, taxonomic, and environmental significance: Palaeogeography, Palaeoclimatology, Palaeoecology, v. 23, p. 199–229, https://doi.org/10.1016/0031-0182(78)90094-9.
- Genise, J.F., 2016, Ichnoentomology, Insect Traces in Soils and Paleosols: Topics in Geobiology: Amsterdam, Springer, v. 37, 695 p., https://doi.org/10.1007/978-3-319-28210-7.
- Holland, S.M., 2010, Additive diversity partitioning in palaeobiology: Revisiting Sepkoski’s question: Palaeontology, v. 53, p. 1237–1254, https://doi.org/10.1111/j.1475-4983.2010.01017.x.
- Kiessling, W., Aberhan, M., and Villier, L., 2008, Phanerozoic trends in skeletal mineralogy driven by mass extinctions: Nature Geoscience, v. 1, p. 527–530, https://doi.org/10.1038/ngeo251.
- Knaust, D., 2015, Siphonichnidae (new ichnofamily) attributed to the burrowing activity of bivalves: Ichnotaxonomy, behaviour and palaeoenvironmental implications: Earth-Science Reviews, v. 150, p. 497–519, https://doi.org/10.1016/j .earscirev.2015.07.014.
- Kowalewski, M., Kiessling, W., Aberhan, M., Fürsich, F.T., Scarponi, D., Wood, S.L.B., and Hoffmeister, A.P., 2006, Ecological, taxonomic, and taphonomic components of the post-Paleozoic increase in sample-level species diversity of marine benthos: Paleobiology, v. 32, p. 533–561, https://doi.org/10.1666/05074.1.
- Labandeira, C.C., and Sepkoski, J.J., 1993, Insect diversity in the fossil record: Science, v. 261, p. 310–315, https://doi.org/10.1126/science.11536548.
- Labandeira, C.C., Feng, Z., Rößler, R., Śipiński, A., and Wang, J., 2017, The Permian origin of wood-boring beetles and their ecological context: Geological Society of America Abstracts with Programs, v. 49, no. 6, https://doi.org/10.1130/abs/2017AM-296643.
- Mángano, M.G., and Buatois, L.A., 2014, Decoupling of body-plan diversification and ecological structuring during the Ediacaran–Cambrian transition: Evolutionary and geobiological feedbacks: Proceedings of the Royal Society, Biological Sciences, v. 281, 20140038, https://doi.org/10.1098/rspb.2014.0038.
- Mángano, M.G., and Droser, M., 2004, The ichnologic record of the Ordovician radiation, in Webby, B.D., Droser, M.L., Paris, F., and Percival, I.G., eds., The Great Ordovician Biodiversification Event: New York, Columbia University Press, p. 369–379, https://doi.org/10.7312/webb12678-035.
- Mángano, M.G., Buatois, L.A., Wilson, M.A., and Droser, M.L., 2016, The Great Ordovician Biodiversification Event, in Mángano, M.G., and Buatois, L.A., eds., The trace-fossil record of major evolutionary changes, vol. 1: Precambrian and Paleozoic: Amsterdam, Springer, Topics in Geobiology, v. 39, p. 127–156.
- Miller, A.I., 2009, The consensus that changed the paleobiological world, in Sepkoski, D., and Ruse, M., eds., The Paleobiological Revolution. Essays on the Growth of Modern Paleontology: Chicago, The University of Chicago Press, p. 364–382, https://doi.org/10.7208/chicago/9780226748 597.003.0019.
- Miller, A.I., and Connolly, S.R., 2001, Substrate affinities of higher taxa and the Ordovician Radiation: Paleobiology, v. 27, p. 768–778, https://doi .org/10.1666/0094-373(2001)027<0768: SAOHTA>2.0.CO;2.
- Miller, M.F., and Labandeira, C.C., 2002, Slow crawl across the salinity divide: Delayed colonization of freshwater ecosystems by invertebrates: GSA Today, v. 12, p. 4–10, https://doi.org/10.1130/1052-173(2002)012<0004:SCATSD>2.0.CO;2.
- Minter, N.J., Buatois, L.A., Mángano, M.G., MacNaughton, R.B., Davies, N.S., and Gibling, M.R., 2016a, The prelude to terrestrial invasion event, in Mángano, M.G., and Buatois, L.A., eds., The trace-fossil record of major evolutionary changes, vol. 1: Precambrian and Paleozoic: Amsterdam, Springer, Topics in Geobiology, v. 39, p. 157–204.
- Minter, N.J., Buatois, L.A., Mángano, M.G., Labandeira, C., Davies, N.S., and Gibling, M.R., 2016b, The establishment of continental ecosystems event, in Mángano, M.G., and Buatois, L.A., eds., The trace-fossil record of major evolutionary changes, vol. 1: Precambrian and Paleozoic: Amsterdam, Springer, Topics in Geobiology, v. 39, p. 205–324.
- Minter, N.J., Buatois, L.A., Mángano, M.G., Davies, N.S., Gibling, M.R., MacNaughton, R.B., and Labandeira, C.C., 2017, Early bursts of diversification defined the faunal colonization of land: Nature Ecology & Evolution, v. 1, https://doi.org/10.1038/s41559-017-0175.
- Orr, P.J., 2001, Colonization of the deep-marine environment during the early Phanerozoic: The ichnofaunal record: Geological Journal, v. 36, p. 265–278, https://doi.org/10.1002/gj.891.
- Pemberton, S.G., and Kobluk, D.R., 1978, Oldest known brachiopod burrow: The Lower Cambrian of Labrador: Canadian Journal of Earth Sciences, v. 15, p. 1385–1389, https://doi .org/10.1139/e78-146.
- Plaziat, J.C., and Mahmoudi, M., 1988, Trace fossils attributed to burrowing echinoids: A revision including new ichnogenus and ichnospecies: Geobios, v. 21, p. 209–233, https://doi.org/10.1016/S0016-6995(88)80019-6.
- Radley, J.D., 2010, Grazing bioerosion in Jurassic seas: A neglected factor in the Mesozoic marine revolution?: Historical Biology, v. 22, p. 387–393, https://doi.org/10.1080/08912961003673079.
- Raup, D.M., 1972, Taxonomic diversity during the Phanerozoic: Science, v. 177, p. 1065–1071, https://doi.org/10.1126/science.177.4054.1065.
- Raup, D.M., 1979, Biases in the fossil record of species and genera: Bulletin of the Carnegie Museum of Natural History, v. 13, p. 85–91.
- Seilacher, A., 1974, Flysch trace fossils: Evolution of behavioural diversity in the deep-sea: Neues Jahrbuch für Geologie und Paläontologie Monatshefte, v. 1974, p. 233–245.
- Seilacher, A., 1977, Evolution of trace fossil communities, in Hallam, A., ed., Patterns of Evolution: Amsterdam, Elsevier, p. 359–376.
- Seilacher, A., 1985, Trilobite paleobiology and substrate relationships: Transactions of the Royal Society of Edinburgh, Earth Sciences, v. 76, p. 231–237, https://doi.org/10.1017/S0263593300010464.
- Sepkoski, J.J., 1978, A kinetic model of Phanerozoic taxonomic diversity I. Analysis of marine orders: Paleobiology, v. 4, p. 223–251, https://doi.org/10.1017/S0094837300005972.
- Sepkoski, J.J., 1979, A kinetic model of Phanerozoic taxonomic diversity II. Early Phanerozoic families and multiple equilibria: Paleobiology, v. 5, p. 222–251, https://doi.org/10.1017/S0094837300006539.
- Sepkoski, J.J., 1981, A factor analytic description of the Phanerozoic marine fossil record: Paleobiology, v. 7, p. 36–53, https://doi.org/10.1017/S0094837300003778.
- Sepkoski, J.J., 1984, A kinetic model of Phanerozoic taxonomic diversity III. Post-Paleozoic families and mass extinctions: Paleobiology, v. 10, p. 246–267, https://doi.org/10.1017/S0094837300008186.
- Sepkoski, J.J., 1997, Biodiversity: Past, present, and future: Journal of Paleontology, v. 71, p. 533–539, https://doi.org/10.1017/S0022336000040026.
- Sepkoski, J.J., Bambach, R.K., Raup, D.M., and Valentine, J.W., 1981, Phanerozoic marine diversity: A strong signal from the fossil record: Nature, v. 293, p. 435–437, https://doi.org/10.1038/293435a0.
- Smith, A.B., and Crimes, T.P., 1983, Trace fossils formed by heart urchins: A study of Scolicia and related traces: Lethaia, v. 16, p. 79–92, https://doi .org/10.1111/j.1502-3931.1983.tb01147.x.
- Sperling, E.A., 2013, Tackling the 99%: Can we begin to understand the paleoecology of the small and soft-bodied animal majority?: The Paleontological Society Papers, v. 19, Ecosystem Paleobiology and Geobiology, p. 77–86, https://doi.org/10.1017/S1089332600002692.
- Tapanila, L., 2006, Devonian Entobia borings from Nevada, with a revision of Topsentopsis: Journal of Paleontology, v. 80, p. 760–767, https://doi.org/10.1666/0022-3360(2006)80[760:DEBFNW]2.0 .CO;2.
- Tapanila, L., and Roberts, E.M., 2012, The earliest evidence of Holometabolan insect pupation in conifer wood: PLoS One, v. 7, e31668, https://doi .org/10.1371/journal.pone.0031668.
- Taylor, P.D., and Wilson, M.A., 2003, Palaeoecology and evolution of marine hard substrate communities: Earth-Science Reviews, v. 62, p. 1–103, https://doi.org/10.1016/S0012-8252(02)00131-9.
- Uchman, A., 2004, Phanerozoic history of deep-sea trace fossils, in McIlroy, D., ed., The application of ichnology to palaeoenvironmental and stratigraphic analysis: Geological Society, London, Special Publication 228, p. 125–139.
- Valentine, J.W., 1969, Niche diversity and niche size patterns in marine fossils: Journal of Paleontology, v. 43, p. 905–915.
- Villegas-Martín, J., de Gibert, J.M., Rojas-Consuegra, R., and Belaústegui, Z., 2012, Jurassic Teredolites from Cuba: New trace fossil evidence of early wood-boring behavior in bivalves: Journal of South American Earth Sciences, v. 38, p. 123–128, https://doi .org/10.1016/j.jsames.2012.06.009.
- Wilson, M.A., and Palmer, T.J., 2006, Patterns and processes in the Ordovician bioerosion revolution: Ichnos, v. 13, p. 109–112, https://doi.org/10.1080/10420940600850505.
- Xing, L., Parkinson, A.H., Ran, H., Pirrone, C.A., Roberts, E.M., Zhang, J., Burns, M.E., Wang, T., and Choiniere, J., 2015, The earliest fossil evidence of bone boring by terrestrial invertebrates, examples from China and South Africa: Historical Biology, v. 28, p. 1108–1117, https://doi.org/10.1080/08912963.2015.1111884.