Full Text View
Volume 28 Issue 2 (February 2018)
GSA Today
Article, pp. 4-10 | Abstract | PDF (2.2MB)
Twenty Years of Subduction Zone Science: Subduction Top to Bottom 2 (ST2B-2)
Cover Image
Table of Contents
- Introduction
- What Have we Learned about Subduction in the Past Twenty Years?
- The Makeup of ST2B-2
- Acknowledgments
- References Cited
Search GoogleScholar for
Search GSA Today

Abstract
No other plate-tectonic setting has attracted such diverse, multidisciplinary research as convergent margins. Understanding the dynamics of subduction is particularly important for realistic assessment of associated hazards such as earthquakes, tsunamis, and volcanic eruptions. A number of recent initiatives have been successful in building communities not only to investigate subduction processes, but also to convey knowledge about subduction zone processes to other scientists, students, postdocs, and the broader public. These efforts must include synthesizing and simplifying subduction-zone science for classroom presentations and to help prepare the public for subduction-related disasters.
Tremendous advances over the past 20 years or so have been made in subduction zone science, with increasingly multidisciplinary efforts producing some of the greatest insights. We have initiated a publication effort in the GSA journal Geosphere, with a Themed Issue “Subduction Top to Bottom 2” (or “ST2B-2”) aimed at showcasing the recent advances, following up on the conceptually similar Subduction Top to Bottom published in 1996 as an American Geophysical Union Geophysical Monograph. The ST2B-2 Geosphere Themed Issue is accumulating papers and is open to ALL wishing to contribute to this effort—we anticipate accepting manuscripts through all of 2018 and possibly beyond.
Manuscript received 18 July 2017. Revised manuscript received 30 Sept. 2017. Manuscript accepted 4 Oct. 2017. Posted online 15 Nov. 2017.
© The Geological Society of America, 2017. CC-BY-NC.
Introduction
Subduction is a uniquely powerful and important Earth process, so it is no surprise that the geoscientific community has become increasingly interested in the process since the term was introduced by White et al. (1970; also see the prescient sketch of a subduction zone by Coats, 1962) on the heels of the plate tectonic revolution. Subduction zones are where lithosphere is recycled into the mantle and they provide the third dimension for the ~55,000 km length of convergent plate margins. The sinking of lithosphere in subduction zones provides most of the power for plate motions and is directly responsible for crustal deformation and arc magmatism. Convergent margin processes affect climate directly (volcanic gasses) and indirectly (producing relief and stimulating weathering), contributing importantly to maintaining Earth’s habitability, at the same time producing societal benefits (ore deposits and hydrocarbon-rich basins) and some of the most dangerous natural hazards (earthquakes, tsunamis, and explosive volcanism). In spite of their importance, subduction zones are not easy to study because they are hidden deeply in the Earth; teasing out their secrets has involved years of efforts by geologists, geophysicists, and geochemists from many different countries working on land, at sea, and using observations from space. The enormity of the challenge of studying subduction zones has spurred efforts to build a cross-disciplinary community of government, academic, and industrial geoscientists from many nations, and for members of this community to learn how to explain their findings to scientists with different expertise as well as to students and the public. Workshops and edited volumes play key roles in building this community. An excellent early example of this community-building effort was held 23 years ago, and the success of this workshop and the resultant publication encourages continued efforts along these lines.
“SUBCON” (Subduction Conference) was held in Avalon, Santa Catalina Island, California, USA, on 12–17 June 1994, largely funded by the United States Geological Survey (USGS) but with additional support from the National Science Foundation (NSF). At this conference, ~120 scientists from around the world shared their understanding of subduction zone dynamics as a function of depth—from top to bottom—bringing together observations and predictions from the many diverse perspectives of the group. Although it is now common to see multidisciplinary groups working together to understand subduction, this was not so in the mid-1990s. To many at SUBCON, the audience to which they revealed their work was unusually diverse in interests and expertise, resulting in some surprising and useful feedback. As a part of SUBCON, the group visited selected outcrops of the Catalina Schist exposed on the island and, for many of the participants, this was a first view of an ancient subduction zone. SUBCON and its aftermath helped lead to some very productive multidisciplinary collaborations, including the 1996 publication of Subduction Top to Bottom (Bebout et al., 1996, American Geophysical Union [AGU] Geophysical Monograph 96, sometimes called “Big Purple” because of the cover). Big Purple contains 39 papers of either a review or case-study nature covering all aspects of subduction zones and their products.
In the 20 or so years since SUBCON and Big Purple, many advances have been made in the fields of geophysics, petrology, geochemistry, and geodynamics, with the work increasingly conducted by highly multidisciplinary groups. Understanding of subduction dynamics has greatly benefited from this approach, leading to an increasingly sophisticated view of subduction zones and how they evolve. More comparative studies have also helped to move the community away from thinking that each convergent margin segment is unique and toward seeing subduction as the central problem, with individual margins showing different variations—and thus opportunities for insights—on the unifying theme. As we’ve examined individual margins in greater detail and contrasted them with other margins, patterns have emerged that reveal some of the controls on convergent margin behavior and evolution. In fact, quite a number of modern subduction margins show dramatic along-strike variations in key physical factors influencing their thermal and mechanical evolution. A few of these factors are convergence rate and obliquity; age of incoming plate and the subduction zone itself; physical, thermal, and chemical state of the subducting oceanic lithosphere; presence of seamounts and other heterogeneities on the downgoing plate; the nature and thickness of subducting sediments; accretion versus erosion; and the composition and structure of the upper plate.
Understanding of the dynamics of subduction is of particular importance in assessing the associated hazards of earthquakes, tsunamis, and volcanic eruptions. The scientific community, governments, and the broader public increasingly recognize the need to assess hazards that subduction margins pose, especially to regions of high population densities around the Pacific and Indian Oceans (e.g., Japan, Indonesia, the Cascadia margin). Recent initiatives promoting this effort include community-led NSF initiatives such as MARGINS and its successor GeoPRISMS, in each of which subduction-zone science has constituted a major component. The two initiatives have identified “focus sites” where effort was concentrated, and these included MARGINS Subduction Factory and SEIZE (SEIsmogenic ZonE) efforts in Central America, Izu-Bonin-Mariana, and Nankai, and GeoPRISMS Subduction Cycles and Deformation focus sites of the Cascadia, Aleutian/Alaska, and New Zealand subduction zones. The MARGINS-sponsored Theoretical Institute, “Inside the Subduction Factory,” in 2000 was a particularly important milestone, resulting in AGU Geophysical Monograph 138 (J. Eiler, ed., 2003). Major efforts similar to MARGINS and GeoPRISMS have been undertaken by subduction communities in Japan, Europe, New Zealand, Central and South America, and southeast Asia, resulting in significant investment in subduction zone science by their respective national governments.
This is an exciting time for those interested in understanding subduction margins! In addition to the GeoPRISMS initiative, the U.S. subduction science community is discussing the potential of a “Subduction Zone Observatory,” which is presented in a “SZ4D Initiative” report aimed at revealing the short- and long-term evolution of subduction margins. That report resulted from an NSF-sponsored workshop in 2016 that was attended by 250 scientists from the USA and 22 foreign countries (www.iris.edu/hq/workshops/2016/09/szo_16). The SZ4D Initiative, as presently configured, proposes three key components: a modeling component, an interdisciplinary science program, and a community infrastructure program (see McGuire et al., 2017). Its science “net” is cast widely, with the aim of fostering integrated geophysics, geology, petrology, geochemistry, and geodynamic modeling. Planning for future subduction zone studies is also being undertaken by the USGS, which has recently announced a major directive, “Reducing Risk Where Tectonic Plates Collide—A Plan to Advance Subduction Zone Science” (www.usgs.gov/news/usgs-publishes-a-new-blueprint-can-help-make-subduction-zone-areas-more-resilient; Gomberg et al., 2017). As its name implies, this initiative aims to focus geological, geophysical, and petrologic/geochemical investigations and modeling at understanding and forecasting hazards associated with subduction plate boundaries. Naturally, the Cascadia margin figures prominently in this planned endeavor because of the large earthquake, tsunami, and volcanic hazards it poses to the increasingly populated Pacific Northwest region. Another example of an initiative emphasizing study of subduction processes and hazards is the ZIP project (Zooming in between Plates), which is a collaborative research and training project funded by the European community as a European Marie Curie Initial Training Network (www.zip-itn.eu). This project involves 12 Ph.D. students and two postdoctoral fellows in a network comprising twelve leading international universities and research centers and nine industrial partners. The U.S. GeoPRISMS E-FIRE project (ExTerra Field Institute and Research Endeavor: Western Alps; http://geoprisms.org/exterra/e-fire/), funded by the NSF’s PIRE program (Partnerships in International Research and Education), builds on the success of ZIP, providing support for eight Ph.D. students and two postdoctoral fellows at 10 academic institutions.
What Have we Learned about Subduction in the Past Twenty Years?
As a thought exercise for this report, each of the co-authors assembled a list of what they consider to be the greatest advancements in subduction zone science in the past two decades since SUBCON/Big Purple. Several themes were repeated on our lists and we agreed on the following. This list can of course be quibbled with, but it does capture how exciting subduction science has been and the range of approaches that are being used and the diverse research communities that have been involved:
Some Advances in Subduction Zone Science in the Past 20 Years
- Improved understanding of how new subduction zones form (see the review by Stern and Gerya, 2018);
- The importance of outer rise normal faulting and deep hydration of subducted lithospheric mantle, and the connection with deep-seated seismicity (e.g., Ranero et al., 2005; Van Avendonk et al., 2011; Fig. 1);
- Understanding the magnitude and significance of subduction erosion; that most convergent margins lose material from the upper plate and only a minority add material by growing accretionary prisms (e.g., Scholl and von Huene, 2007);
- Exploration of submarine arc and backarc basin volcanoes and associated hydrothermal systems and volcaniclastic sedimentation, especially in the Izu-Bonin-Mariana and Tonga Kermadec systems (e.g., Baker et al., 2008; Dziak et al., 2015);
- Recent major subduction earthquakes (such as the 2004 Sumatra and the 2011 Tohoku-Oki earthquakes) have taught us important lessons about what is possible in these great events. For example, completely unexpected and massive near-trench slip (up to 50 m) in the 2011 Tohoku-Oki earthquake (Fujiwara et al., 2011) has important implications for tsunamigenesis and the nature of slip near the trench;
- Discovery of slow-slip events in subduction zones, and the spectrum of seismological and geodetic phenomena related to slow slip (see Fig, 2, which is from Gomberg et al., 2010). Densification of continuous GPS networks above many subduction zones has been especially important in this effort (see the discussion by Gomberg et al., 2017);
- Statistical and observational documentation that high-magnitude megathrust earthquakes may be linked to where wide expanses of thick sediment and bathymetrically smooth seafloor enter subduction zones (e.g., Brizzi et al., 2018), while subduction margins with rough incoming plates and low sedimentary thicknesses appear to be dominated by aseismic creep and more moderate-sized earthquakes (Wang and Bilek, 2011);
- Increased appreciation of the role that fluids play in subduction margin mechanics and seismogenesis (Saffer and Tobin, 2011);
- Seismological advances that better resolve earthquake structure and mechanisms in the downgoing plate (e.g., Rondenay et al., 2008; Shillington et al., 2015) and improved tomography to image the subducted slab at depths greater than the 670 km limit of earthquakes (van der Hilst et al., 1997);
- Accelerating exploration of the deep oceanic trenches because of technological advances in manned submersibles, remotely operated vehicles, and autonomous undersea vehicles (e.g., Cui et al., 2013; Okumura et al., 2016);
- Massively increased computational power allowing corresponding advances in numerical modeling of subduction zone thermal structure, metamorphism, rheology, and chemical budgets (e.g., van Keken et al., 2011; Hacker and Gerya, 2013; see Figs. 3 and 4);
- Greater understanding of connections between studies of exhumed paleo-subduction complexes (high- and ultrahigh-pressure rocks) and processes in active subduction zones, revealing intricacies between short- (seconds) and long-term (million years) deformation on plate interfaces (see Figs. 4 and 5; Angiboust et al., 2012a, 2012b), the volumetric importance of subcrustal accretionary underplating (e.g., Bassett et al., 2010) versus frontal accretion, as well as providing insights about chemical cycling in and above subduction zones (see the review by Bebout, 2014, and references therein);
- Studies of ultrahigh-pressure metamorphic rocks, coupled with thermomechanical models, demonstrating that oceanic and continental crust can be subducted to >100 km depth and returned to the surface (e.g., Gerya et al., 2002; Yamato et al., 2008);
- Improved understanding of the nature of supercritical fluids, where they exist in and above subduction zones, and their mass transport capabilities (via experimental and theoretical approaches; e.g., Manning, 2004; Hermann et al., 2006), and appreciation of the tremendous amounts of subducted water that could be stored in the mantle transition zone;
- Microanalytical advances allowing measurement of volatiles and trace element contents in minerals and melt inclusions, further constraining chemical cycling through subduction zones (Frezzotti et al., 2011) and the causes of explosive arc volcanism (e.g., Wallace, 2005; Zellmer et al., 2015);
- Improved understanding of chemical recycling via subduction of oceanic crust, sediment, and uppermost mantle (e.g., Plank, 2005), especially the cycling of volatiles at convergent margins (e.g., Hilton et al., 2002; Mason et al., 2017) and technical advances enabling field measurements of arc volcanic gas emissions (e.g., Fischer and Lopez, 2016).
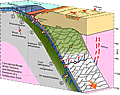
Cartoon showing a conceptual model of the structure and metamorphic evolution of subducting lithosphere formed at a fast spreading center (from Ranero et al., 2005). The topography of the plate in the outer-rise/trench region has been exaggerated to better show the deformation associated with plate bending. Scale is otherwise approximately correct. Fault plane solutions of earthquakes are projected into the top of the slab and the plane of the cross section. The small black-filled circles in oceanic crust indicate hydration.
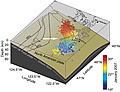
Illustration of northern 2007 episodic tremor and slip (ETS) event in Cascadia (from Gomberg et al., 2010). The oceanic Juan de Fuca plate subducts beneath the North America plate at ~4 cm/yr roughly perpendicular to the coast (white arrow). The plates are coupled for part of their interface (tan-colored surface) such that relative motion is inhibited or “locked” to a varying degree. Uncertain are the location and mechanism by which the locking changes to a freely slipping interface. The fraction of relative plate motion is portrayed as continuous aseismic slip that increases down-dip from 40% to 80% (dashed contours). Inland of the locked zone, tremor epicenters projected onto the plate interface (circles) overlie the area that experienced slow slip (gray area on plate interface) during the last two weeks of January 2007. Color shading of tremor epicenters shows its temporal migration.
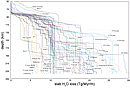
Diverse H2O loss calculated as a function of depth for oceanic lithosphere and sediment subducting into each of Earth’s modern subduction zones (the “Tokyo Subway Map” from van Keken et al., 2011). The warmest subduction zones lose most of their H2O beneath the forearc. All subducting slabs lose significant water when the slab comes into contact with the hot overlying mantle wedge (in these models, at 80 km depth). For many slabs (e.g., Kamchatka, Calabria) further dehydration is minor. Other slabs (e.g., Chile) continue to dehydrate significantly with increasing depth principally due to the dehydration of the uppermost mantle. A few slabs (e.g., Marianas) are very cool, and far less H2O is lost to even 230 km depths.
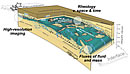
Schematic view of a subduction zone between 35 and 85 km depth based on numerical model results (and on study of exhumed/exposed ophiolitic terranes) showing inferred morphologies and the detachment of large folded slices of oceanic lithosphere, accreted along the plate interface (from Angiboust et al., 2012b). This figure also illustrates the main deformation-enhanced fluid pathways (associated with deep serpentinite producing/consuming reactions), dominantly at the boundary between materials with marked rheological contrasts.
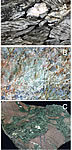
Examples of brecciation and fault-related melting of subduction-zone metamorphic rocks (the photographs in [A] and [C] are from Samuel Angiboust, personal commun., 2017; the photograph in [B] is from Michele Locatelli, personal commun., 2017). (A) Pseudotachylite (result of fault-related frictional heating) from the Arosa Zone St. Moritz (Switzerland) exposure of ~35 km depths of an ancient subduction zone interface investigated by Bachmann et al. (2009). The horizontal dimension is 5 cm. In this photograph, we see the result of plastic deformation (clasts are mylonitized) and evidence of melt injection during the seismic event. (B) Eclogite-facies breccia from the Monviso ultra-high pressure (UHP) metamorphic locality (Italy) representing a major rupture event at ~80 km depth in an ancient subduction zone (the horizontal dimension is ~1.0 m; see Angiboust et al., 2012a). (C) Another example of intensely fractured/brecciated eclogite-facies metabasaltic rock from the UHP metamorphic Monviso locality, showing the mineralization of these fractures by omphacite, a Na-rich pyroxene stable at extremely high pressure-temperature (P/T) conditions (the horizontal dimension is 15 cm). The mineralized matrix of the breccia in (B), and the veins in (C), reflect high-P/T element mobility, likely in H2O-rich metamorphic fluids.
Now, ~20 years after SUBCON and Big Purple, we feel it is the right time to put together another dedicated volume highlighting these major breakthroughs. In this effort, we return to the philosophy of previous ventures for an updated volume called “Subduction Top to Bottom 2,” or ST2B-2 for short, that is now soliciting manuscripts. The goal of the ST2B-2 Geosphere special issue is to assemble a large number of papers arranged by the subduction-zone depth horizon they consider, again, independent of the methods used. We particularly encourage manuscripts employing diverse observations and methods to identify problems where differing disciplines examine similar processes. To raise awareness of the new volume, we have been holding “Subduction Top to Bottom 2” sessions (Fall 2016 AGU and 2017 GSA Annual and Fall AGU meetings).
As examples of where multidisciplinary pursuits could be particularly fruitful, forearc seismic events commonly originate along the active subduction interface or in accreted sediments experiencing pressure-temperature conditions preserved in forearc metasedimentary suites representing similar but ancient processes. Might examination of the metamorphic rock record better tell us how slow slip events and related seismic phenomena (such as tremor) happen (see Fig. 2) and, more specifically, the roles of fluids in generating such events? Could highly brecciated zones of eclogite from ancient subduction zones be the products of catastrophic energy release along the interface that generated ancient earthquakes (see Fig. 5)? Could laboratory experiments regarding the rheology and mechanical failure of rocks lead to greater understanding of the relationships between devolatilization and other metamorphic reactions and observed seismicity (e.g., Incel et al., 2017)? Can we decipher the balance of material delivered through an individual subduction margin by combining knowledge of inputs derived through deep-sea drilling, forearc heat flow measurements, thermal modeling, thermodynamic calculations, analysis of ancient metamorphic rocks, and analyses of volcanic gases?
The Makeup of ST2B-2
The ST2B-2 endeavor is intended to generate a large, online themed issue in the Geological Society of America journal Geosphere. With this online format—in our view a clear example of the future of scientific communication—we are unencumbered by page limits imposed by a physical book and by costs of color figures; furthermore, this format encourages use of interactive graphics and online data sets. Individual papers are published soon after manuscripts are accepted; there is no waiting for the slowest author(s). Published works in Geosphere are landscape-format and so more amenable to viewing on a computer screen. For comparison, Big Purple faced strict manuscript length guidelines, authors did their own formatting (following the old style of AGU journals), resulting in a fairly unpolished appearance, publication of color graphics was expensive (and thus few authors published color graphics), and we had a strict time deadline in order to keep the book publication project on schedule (resulting in some authors ultimately not submitting manuscripts). In the end, Big Purple contained 39 papers, varying greatly in length, with uneven coverage of the full range of top-to-bottom subduction science.
For the all-electronic Geosphere themed issue, we have identified 17 subduction-zone science categories, arrayed as a function of increasing depth in a subduction zone, beginning with “What Goes In” and “Forces Driving Subduction.” Each of these science categories has one to three assistant guest editors (AGEs) assigned to identifying authors invited to contribute manuscripts and be the contact individuals for those wanting to submit manuscripts. The science categories (and the associated AGEs) are as follows—at the time of acceptance of this paper, Geosphere ST2B-2 had amassed ~40 manuscripts in various stages of review, revision, production, and publication.
Outline of ST2B-2 Geosphere Themed Issue
- Introduction;
- What goes in (seafloor lithosphere and sediment, seamounts, and aseismic ridges). AGEs: Mike Underwood and Andy Fisher;
- Forces driving subduction—thermal and geodynamic modeling. AGE: Taras Gerya;
- Getting started (subduction initiation). AGE: Mark Reagan;
- Outer rise (slab bending, deep hydration of slabs). AGEs: Doug Wiens, Cesar Ranero;
- Shallow forearc dynamics (initial dewatering and diagenesis, fluids, accretion, erosion). AGE: Nathan Bangs;
- Deformation of and physical conditions in the subduction interface from the seismogenic zone through the source of episodic slow slip and tremor. AGEs: Shuichi Kodaira, Sue Bilek, and Samuel Angiboust;
- Upper plate deformation over varying timescales. AGEs: Frédérique Leclerc and Nathalie Feuillet;
- Into the pressure cooker (metamorphism, fluid-rock interactions, records of deep underplating and exhumation, nature of deep subduction interface; also including arc delamination and drips). AGEs: Sarah Penniston-Dorland and Ake Fagereng;
- Forearc to subarc mantle wedge. AGEs: Maureen Long and Marco Scambelluri;
- Subduction zone magmatism (models for evolution, petrology, geochemistry, and isotopes, including batholiths). AGE: Paul Wallace;
- Explosive volcanism hazards. AGE: Bob Tilling;
- Geochemical and seismological expressions of deep subducted slabs. AGEs: Catherine Chauvel and Stéphane Rondenay;
- Backarc basins, cross chains, and fold-and-thrust belts. AGEs: Fernando Martinez and Ron Hyndman;
- Resource implications. AGEs: Gray Bebout, Bob Stern, and Dave Scholl;
- Crust formation at convergent margins. AGEs: Kiyoshi Suyehiro and Kent Condie; and
- Convergent margin education and outreach. AGE: Bob Stern.
The Geosphere ST2B-2 themed issue can be accessed at pubs.geoscienceworld.org/geosphere/pages/st2b2. It is open to ALL wishing to contribute to this effort. Ideally, papers in the issue will cover each of the 17 topics, and we are optimistic that more than 100 papers will ultimately be published. We anticipate that submissions for the ST2B-2 themed issue will be accepted at the least through the end of 2018, and we encourage anyone interested in contributing to contact either one of the five guest editors (one of the five of us) or the AGE(s) associated with the science category into which you envision your contribution fitting.
Acknowledgments
We thank Shan de Silva, science editor of Geosphere, for working with us to plan and coordinate the assembly of this themed issue. Many thanks to reviewer Peter Kelemen for his constructive input, particularly in the assembly of the list of “Some Advances in Subduction Zone Science in the Past 20 Years,” to the other, anonymous reviewer, and to Steve Whitmeyer for his efforts as GSA Today editor.
References Cited
- Angiboust, S., Agard, P., Yamato, P., and Raimbourg, H., 2012a, Eclogite breccias in a subducted ophiolite: A record of intermediate-depth earthquakes?: Geology, v. 40, p. 707–710, https://doi.org/10.1130/G32925.1.
- Angiboust, S., Wolf, S., Burov, E., Agard, P., and Yamato, P., 2012b, Effect of fluid circulation on subduction interface tectonic processes: Insights from thermo-mechanical numerical modeling: Earth and Planetary Science Letters, v. 357–358, p. 238–248, https://doi.org/10.1016/j.epsl.2012.09.012.
- Bachmann, R., Oncken, O., Glodny, J., Seifert, W., Georgieva, V., and Sudo, M., 2009, Exposed plate interface in the European Alps reveals fabric styles and gradients related to an ancient seismogenic coupling zone: Journal of Geophysical Research, v. 114, B05402, https://doi.org/10.1029/2008JB005927.
- Baker, E.T., Embley, R.W., Walker, S.L., Resing, J.A., Lupton, J.E., Nakamura, K.-I., de Ronde, C.E.J., and Massoth, G.J., 2008, Hydrothermal activity and volcano distribution along the Mariana arc: Journal of Geophysical Research, v. 113, B08S09, https://doi.org/10.1029/2007JB005423.
- Bassett, D., Sutherland, R., Henrys, S., Stern, T., Scherwath, M., Benson, A., Toulmin, S., and Herderson, M., 2010, Three-dimensional velocity structure of the northern Hikurangi margin, Raukuara, New Zealand: Implications for the growth of continental crust by subduction erosion: Geochemistry Geophysics Geosystems, v. 11, no. 10, Q10013, https://doi.org/10.1029/2010GC003137.
- Bebout, G.E., 2014, 4.20 Chemical and isotopic cycling in subduction zones: Treatise on Geochemistry, v. 4, p. 703–747, https://doi.org/10.1016/B978-0-08-095975-7.00322-3.
- Bebout, G.E., Scholl, D.W., Kirby, S.H., and Platt, J.P., editors, 1996, Subduction Top to Bottom: Washington, D.C., American Geophysical Union Geophysical Monograph 96, 384 p., https://doi.org/10.1029/GM096.
- Brizzi, S., Sandri, L., Funiciello, F., Corbi, F., Piromallo, C., and Heuret, A., 2018, Multivariate statistical analysis to investigate subduction zone parameters favoring the occurrence of great earthquakes: Tectonophysics (in press).
- Coats, R.R., 1962, Magma type and crustal structure in the Aleutian arc, in Macdonald, G.A., and Kuno, H., eds., The Crust of the Pacific Basin: Washington, D.C., American Geophysical Union Geophysical Monograph 6, p. 92–109, https://doi.org/10.1029/GM006p0092.
- Cui, W., Feng, L., Hu, Z., Min, G., and Liu, C., 2013, On the 7,000 m sea trials of the submersible Jialong: Marine Technology Society, v. 47, p. 67–82, https://doi.org/10.4031/MTSJ.47.1.2.
- Dziak, R.P., Bohnenstiehl, D.R., Baker, E.T., Matsumoto, H., Caplan-Auerbach, J., Embley, R.W., Merle, S.G., Walker, S.L., Lau, T.-K., and Chadwick, W.W., Jr., 2015, Long-term explosive degassing and debris flow activity at West Mata submarine volcano: Geophysical Research Letters, v. 42, p. 1480–1487, https://doi.org/10.1002/2014GL062603.
- Eiler, J., editor, 2003, Inside the Subduction Factory: Washington, D.C., American Geophysical Union Geophysical Monograph 138, 311 p., https://doi.org/10.1029/GM138.
- Fischer, T.P., and Lopez, T.M., 2016, First airborne samples of a volcanic plume for d13C of CO2 determinations: Geophysical Research Letters, https://doi.org/10.1002/2016GL068499.
- Frezzotti, M.L., Selverstone, J., Sharp, Z.D., and Compagnoni, R., 2011, Carbonate dissolution during subduction revealed by diamond-bearing rocks from the Alps: Nature Geoscience, v. 4, p. 703–706, https://doi.org/10.1038/ngeo1246.
- Fujiwara, T., Kodaira, S., No, T., Kaiho, Y., Takahashi, N., and Kaneda, Y., 2011, The 2011 Tohoku-oki earthquake: Displacement reaching the trench axis: Science, v. 334, 1240, https://doi.org/10.1126/science.1211554.
- Gerya, T.V., Stockhert, B., and Perchuk, A.L., 2002, Exhumation of high-pressure metamorphic rocks in a subduction channel: A numerical simulation: Tectonics, v. 21, no. 6, 1056, https://doi.org/10.1029/2002TC001406.
- Gomberg, J., and the Cascadia 2017 and Beyond Working Group, 2010, Slow-slip phenomena in Cascadia from 2007 and beyond: A review: Geological Society of America Bulletin, v. 122, p. 963–978, https://doi.org/10.1130/B30287.1.
- Gomberg, J.S., and 17 others, 2017, Reducing risk where tectonic plates collide—A plan to advance subduction zone science: United States Geological Survey Circular 1428, 45 p., https://doi.org/10.3133/cir1428.
- Hacker, B.R., and Gerya, T.V., 2013, Paradigms, new and old, for ultrahigh-pressure tectonism: Tectonophysics, v. 603, p. 79–88, https://doi.org/10.1016/j.tecto.2013.05.026.
- Hermann, J., Spandler, C., Hack, A., and Korsakov, A.V., 2006, Aqueous fluids and hydrous melts in high-pressure and ultra-high pressure rocks: Implications for element transfer in subduction zones: Lithos, v. 92, p. 399–417, https://doi.org/10.1016/j.lithos.2006.03.055.
- Hilton, D.R., Fischer, T.P., and Marty, B., 2002, Noble gases and volatile recycling at subduction zones: Reviews in Mineralogy and Geochemistry, v. 47, no. 1, p. 319–370, https://doi.org/10.2138/rmg.2002.47.9.
- Incel, S., Hilairet, N., Labrousse, L., John, T., Deldicque, D., Ferrand, T., Wang, Y., Renner, J., Morales, L., and Schubnel, A., 2017, Laboratory earthquakes triggered during eclogitization of lawsonite-bearing blueschist: Earth and Planetary Science Letters, v. 459, p. 320–331, https://doi.org/10.1016/j.epsl.2016.11.047.
- Manning, C.E., 2004, The chemistry of subduction-zone fluids: Earth and Planetary Science Letters, v. 223, p. 1–16, https://doi.org/10.1016/j.epsl
- .2004.04.030.
- Mason, E., Edmonds, M., and Turchyn, A.V., 2017, Remobilization of crustal carbon may dominate volcanic arc emissions: Science, v. 357, p. 290–294, https://doi.org/10.1126/science.aan5049.
- McGuire, J., Plank, T., et al., (2017) The SZ4D Initiative: Understanding the Processes that Underlie Subduction Zone Hazards in 4D: The IRIS Consortium, 63 p., https://www.iris.edu/hq/files/workshops/2016/09/szo_16/sz4d.pdf.
- Okumura, T., Watanabe, H., Chen, C., Ohnishi, Y., Yamanaka, T., Sakai, S., Bloomer, S.H., Pujana, I., Stern, R.J., Ishii, T., Takai, K., and Ohara, Y., 2016, The world’s deepest brucite-carbonate chimneys at a serpentinite-hosted system, the Shinkai Seep Field, Southern Mariana Forearc: Geochemistry Geophysics Geosystems, v. 17, https://doi.org/10.1002/2016GC006449.
- Plank, T., 2005, Constraints from thorium/lanthanum on sediment cycling at subduction zones and evolution of the continents: Journal of Petrology, v. 46, p. 921–944, https://doi.org/10.1093/petrology/egi005.
- Ranero, C.R., Villasenor, A., Phipps Morgan, J., and Weinrebe, W., 2005, Relationship between bend-faulting at trenches and intermediate-depth seismicity: Geochemistry Geophysics Geosystems, v. 6, https://doi.org/10.1029/2005GC000997.
- Rondenay, S., Abers, G.A., and van Keken, P.E., 2008, Seismic imaging of subduction zone metamorphism: Geology, v. 36, p. 275–278, doi:https://doi.org/10.1130/G24112A.1.
- Saffer, D.M., and Tobin, H.J., 2011, Hydrogeology and mechanics of subduction zone forearcs: Fluid flow and pore pressures: Annual Review of Earth and Planetary Sciences, v. 39, p. 157–186, https://doi.org/10.1146/annurev-earth-040610-133408.
- Scholl, D.W., and von Huene, R., 2007, Crustal recycling at modern subduction zones applied to the past—Issues of growth and preservation of continental basement crust, mantle geochemistry, and supercontinent reconstruction, in Hatcher, R.D., Jr., Carlson, M.P., McBride, J.H., and Martínez Catalán, J.R., eds., 4-D Framework of Continental Crust: Geological Society of America Memoir 200, p. 9–32, https://doi.org/ 10.1130/2007.1200(02).
- Shillington, D.J., Bécel, A., Nedimović, M.R., Kuehn, H., Webb, S.C., Abers, G.A., Keranen, K.M., Li, J., Delescluse, M., and Mattei-Salicrup, G.A., 2015, Link between plate fabric, hydration and subduction zone seismicity in Alaska: Nature Geoscience, https://doi.org/10.1038/ngeo2586.
- Stern, R.J., and Gerya, T., 2018, Subduction initiation in nature and models: A review: Tectonophysics (in press).
- Van Avendonk, H.J.A., Holbrook, W.S., Lizarralde, D., and Denyer, P., 2011, Structure and serpentinization of the subducting Cocos plate offshore Nicaragua and Costa Rica: Geochemistry Geophysics Geosystems, v. 12, Q06009, https://doi.org/10.1029/2011GC003592.
- van der Hilst, R.D., Widyantoro, S., and Engdahl, E.R., 1997, Evidence for deep mantle circulation from global tomography: Nature, v. 386, p. 578–584, https://doi.org/10.1038/386578a0.
- van Keken, P.E., Hacker, B.R., Syracuse, E.M., and Abers, G.A., 2011, Subduction factory: 4. Depth-dependent flux of H2O from subducting slabs worldwide: Journal of Geophysical Research, v. 116, https://doi.org/10.1029/2010JB007922.
- Wallace, P.J., 2005, Volatiles in subduction zone magmas: Concentrations and fluxes based on melt inclusion and volcanic gas data: Journal of Volcanology and Geothermal Research, v. 140, no. 1–3, p. 217–240, https://doi.org/10.1016/j.jvolgeores.2004.07.023.
- Wang, K., and Bilek, S.L., 2011, Do subducting seamounts generate or stop large earthquakes?: Geology, v. 39, p. 819–822, https://doi.org/10.1130/G31856.1.
- White, D.A., Roeder, D.H., Nelson, T.H., and Crowell, J.C., 1970, Subduction: Geological Society of America Bulletin, v. 81, p. 3431–3432, https:// doi. org/10.1130/0016- 7606(1970)81[3431:S]2.0.CO;2.
- Yamato, P., Burov, E., Agard, P., Le Pourhiet, L., and Jolivet, L., 2008, HP-UHP exhumation during slow continental subduction: Self-consistent thermodynamic and thermomechanically coupled model with application to the Western Alps: Earth and Planetary Science Letters, v. 271, p. 63–74, https://doi.org/10.1016/j.epsl.2008.03.049.
- Zellmer, G.F., Edmonds, M., and Straub, S.M., eds., 2015. The role of volatiles in the genesis, evolution and eruption of arc magmas: Geological Society, London, Special Publication 410, http://dx.doi.org/10.1144/SP410.13.